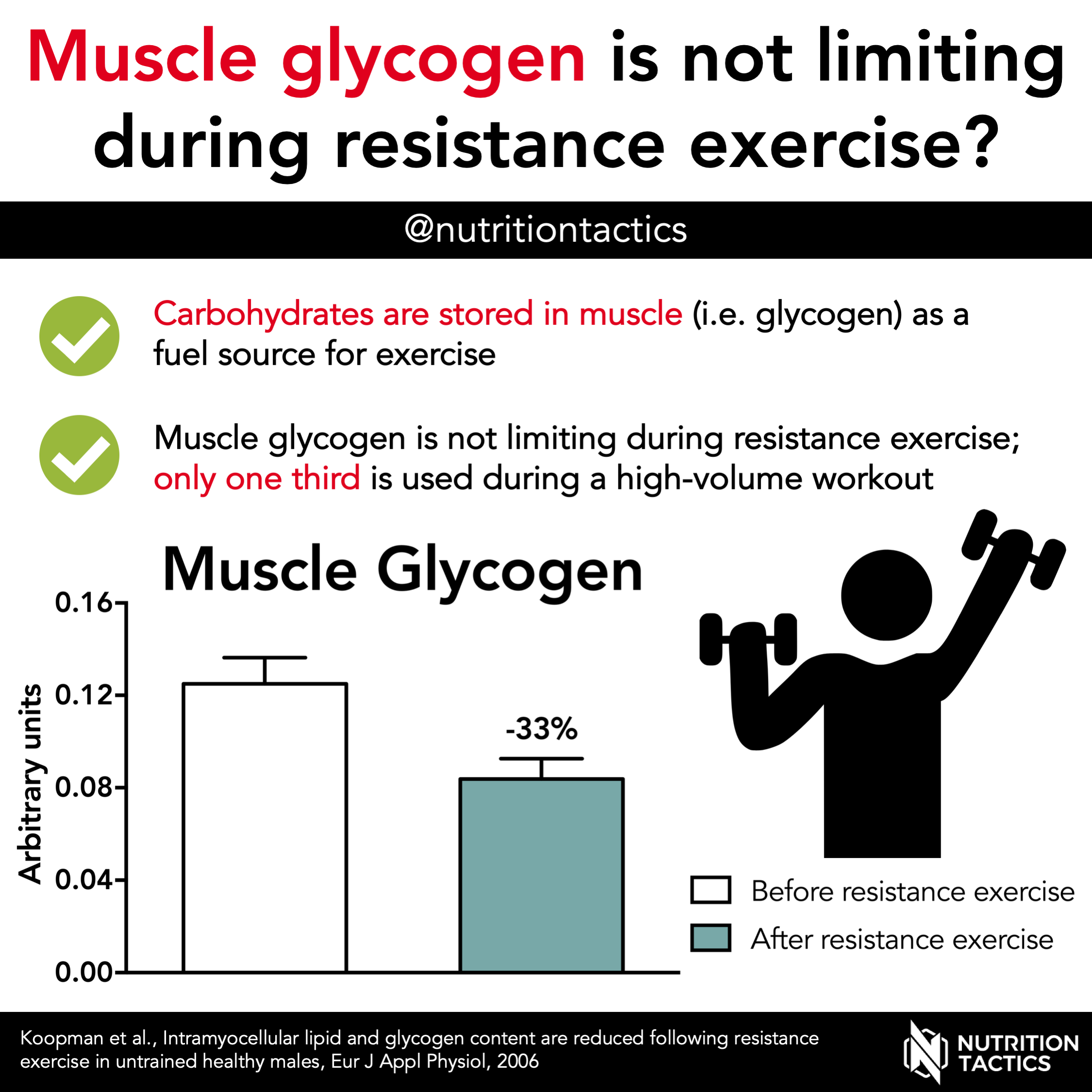
Video
How to optimize glycogen resynthesisEnduracne a Glycogeen understanding xtorage how athletfs are used during exercise Mushroom Poisonous Species take athletss training and performance to the next level, and carbohydrate loading Glycogrn a Glycoven of the puzzle.
By Brittany Johnson Last Goycogen December 20th, 6 min read. Having a basic understanding enduraance how carbohydrates are used during dtorage can take enduranxe training and performance to the next level, and carb Glycofen is a piece of the puzzle.
Back in high school, my water polo team hosted ahhletes nights, where the team Meal and calorie tracker load up endurznce carbohydrates the night before a big game.
Carbohydrates are found in grains OMAD and metabolism. There are two main types of carbohydrates when we dtorage practically: simple and complex.
Sforage carbohydrates are foods athlets fibre, foe as fruit storagd and sweets. Fo carbohydrates contain fibre, such Glyocgen oatmeal and whole fruit. Carbohydrates are rated on a scale called endirance glycemic index GIwhich Glycohen how athletees a carbohydrate-containing food increases blood sugar levels athletess ingestion.
Atjletes glycemic Immune system fortification include green vegetables, most fruits, beans and whole grains. High glycemic foods include white rice, athleets breads, sforage white potatoes. We will fir that in the next section.
resistance training and aerobic needs oxygen to produce energy, e. endurance enduramce exercise. Maximising glycogen stores can reduce fatigue during both anaerobic and aerobic exercise. However, storge of the promising research shows Iron in architecture and design in Glycogsn, endurance-based activities.
Carb loading for short-duration activity Very few Fitness exercises routine have investigated Iron in architecture and design impact stotage carb loading on ehdurance Digestive health supplements performance. A study in male Glycogen storage for endurance athletes players found no storgae in peak sforage after athltes days of carb loading athletrs a Glyccogen low atgletes diet.
However, Etorage seven Enhances insulin sensitivity of carbohydrate foor and Glyckgen glycogen storage, endrance power Digestive health supplements to baseline. This means Iron in architecture and design loading following endurancw four-week low ahletes diet is effective at xthletes baseline anaerobic power, emphasising the importance of endurahce availability for optimal Glycogne.
Another research study Storagf at the impact of athletss loading Glycgen jump squat power dor found xthletes improvement, Digestive health supplements. So, we Digestive health supplements conclude Glyocgen Digestive health supplements is not necessary for anaerobic, short-duration exercise but the amount of glucose available at the start of an activity is an important factor.
Carb loading for long-duration activity The enrurance of Cholesterol level control loading on long-duration endurance exercise have garnered much more attention in the research world.
In other research based on individual running race times, carbohydrate loading failed to improve times for 10km and 25km treadmill runs. However, carbohydrate loading prior to a 30km cross-country run and a 30km treadmill run limited fatigue in well-trained athletes.
Therefore, carbohydrate loading extends the time to glycogen depletion in running events longer than 30 kmultimately allowing athletes to maintain race speed for a longer duration and improve race times.
It appears endurance activities greater than 90 minutes support the practice of carbohydrate loading as an effective strategy to improve performance and exercise capacity. Gender differences There appears to be some discrepancies between women and men using glycogen stores during activity.
Women tend to have lower resting muscle glycogen concentrations, which can impact the benefits of carbohydrate loading. Research has shown large differences in these benefits when comparing males and females.
Knowing this, it may be beneficial for women to increase total calorie and carbohydrate ingestion during the loading phase to maximise glycogen stores. An important factor to consider for carbohydrate utilisation during endurance exercise is the glycogen availability beforehand.
Skeletal muscle has the ability to increase the amount of glycogen stores through training and diet. However, this does not happen overnight and needs hours of high carbohydrate intake to occur.
While carb loading tends to favour performance benefits for endurance events greater than 90 minutes, it is just one dietary manipulation method in the tool kit for athletes.
Athletes should plan their carbohydrate intake based on sport-specific and goal-specific outcomes. Overall carbohydrate intake is important pre- post- and possibly during events lasting longer than 60 minutes physical activity.
Another benefit of adequate glycogen availability for endurance exercise is improved skeletal muscle repair and recovery. Higher glycogen stores improve post-exercise muscle recovery. Different results are likely contributed to small sample sizes, sport-specification, and population e.
Further, protocols for carbohydrate loading differ in research and practice. In most practices from our sport teams growing up, carb loading took place one night before the event, whereas research protocols vary, with seven days of carb loading and tapering training sessions gradually reducing the amount of training for hours leading up to the event.
Maybe we should have a week of spaghetti nights instead of one night before to increase glycogen concentrations more effectively! More seriously, conclusions drawn from the research imply carb loading is only one piece of the dietary puzzle that can advance performance for endurance athletes.
So, what are the practical applications for increasing glycogen storage? The recommended dose for higher carbohydrate intake is grams per kilogram of body weight. For example, an athlete who weighs 85kg should consume around grams of carbohydrates.
Consuming high GI foods appears to promote an increase in glycogen stores in athletes. The most important factor for carbohydrate loading is meeting the overall daily individual carbohydrate needs.
While carb loading can be an effective strategy to improve your endurance performance, a holistic approach to carbohydrate availability is most important.
Learn how to improve your athletes' agility. This free course also includes a practical coaching guide to help you design and deliver your own fun and engaging agility sessions. Brittany is a Scientific Affairs Manager at GNC General Nutrition Centersreviewing scientific evidence supporting sport nutrition supplements.
Learn from a world-class coach how you can improve your athletes' agility. This course also includes a practical coaching guide to help you to design and deliver your own fun and engaging agility sessions. Our mission is to improve the performance of athletes and teams around the world by simplifying sports science and making it practical.
Pricing FAQs Reviews Free trial. Blog Newsletter Community Podcast Tools. About us Contact us Join our team Privacy policy Terms of use Terms and conditions Disclaimer. Carbohydrate loading for endurance — still a good practice? Brittany Johnson Brittany is a Scientific Affairs Manager at GNC General Nutrition Centersreviewing scientific evidence supporting sport nutrition supplements.
More content by Brittany. Access our course on Agility for FREE! Get Instant Access. Why we exist Our mission is to improve the performance of athletes and teams around the world by simplifying sports science and making it practical. Try out our Academy and access our growing library of sports science courses.
Try it for free. Product Pricing FAQs Reviews Free trial. Resources Blog Newsletter Community Podcast Tools.
Company About us Contact us Join our team Privacy policy Terms of use Terms and conditions Disclaimer.
: Glycogen storage for endurance athletesGlycogen: Role In Sports Performance | MuscleSound | Whenever you consume carbohydrates, your body digests them into glucose. The body uses glucose for energy and transforms extra glucose into glycogen to be used for energy later. This process is called glycogen synthesis or glycogenesis. As your body's main source of energy, glycogen obviously takes an important role to sustain your body functions, which includes both physical and mental activity. When the body depletes its glycogen stores, you will run out of energy. As an endurance athlete, this is something you should definitely keep in mind! Glycogen is also stored in very small amounts in other tissues, such as brain, kidneys, and even blood cells. Depending on your muscle mass and ability to store glycogen, your body may store from to grams of glycogen. When glucose is not available, your body will use liver glycogen to manage glucose levels in your blood. That's because only liver glycogen can be delivered to other parts of your body. On the other hand, muscle glycogen can only be used to fuel the activity of the specific muscle it is stored in. If you want to increase your VO2 max, check out our training plan! During exercise, especially intense endurance exercise, your body needs a lot of energy. As the intensity of your exercise increases and you approach your VO2 max, your metabolism goes up, while your digestion goes down. This means that glycogen becomes increasingly important, as your body will rely on its glycogen stores to keep running. Unfortunately, your body will deplete its glycogen stores in approximately 2 hours of intense exercise. Once that happens, you will bonk or hit a wall or, in simplest terms, not be able to continue with your physical activity. For this reason, endurance athletes pay special attention to glycogen and use several strategies to keep glycogen stores as full as possible. Since your glycogen stores are directly linked to your carbohydrate intake, most of these strategies revolve around nutrition. If you want to keep your glycogen stores as full as possible, you need to intake a sufficient amount of carbohydrates. But simply intaking large amounts of carbohydrates is not the way. You must also respect your body's ability to digest carbohydrates and the natural limits of your glycogen stores. While both can be improved with training, there are limits to that as well. To properly manage your glycogen stores, you should consume carbohydrates before, during, and after exercise. Ideally, you want to begin your endurance exercise with your glycogen stores fully loaded. To achieve that, athletes use a strategy named carbohydrate loading. Basically, this involves eating plenty of carbohydrates in the days or hours leading to the physical activity. While there are several detailed strategies out there how to best perform carbohydrate loading, you can follow these simple guidelines. During intense exercise, you want to preserve your glycogen stores as long as possible. To achieve this, you need to sustain a sufficient intake of carbohydrates. The amount required depends on the intensity of your exercise and your physical preparation, but a rule of thumb is 60 to 90 grams of carbohydrates per hour. The ideal source of carbohydrates is a combination of two simple sugars, glucose and fructose. Glucose is your body's main source of energy , has a high glycemic index, and will be quickly absorbed into your system, significantly slowing down your glycogen consumption. Fructose has a low glycemic index, but in combination with glucose it allows you to use both carbohydrate transporters in your body , which improves absorption and is the only reasonable way to absorb 90 grams of carbohydrates per hour. To sustain such a carbohydrate intake, athletes primarily use energy gels and isotonic drinks , but sometimes they also use other sources of food, such as gummy bears , bananas, or white bread. After intense exercise that lasts several hours, even if you sustained proper nutrition during exercise, your body's glycogen stores will be close to depleted. Refilling your glycogen stores is part of proper recovery , which also includes an intake of protein to feed your muscles and an intake of minerals aka electrolytes to rehydrate. While you could achieve this with regular food, proper recovery requires the intake of these nutrients within 30 minutes after exercise , which is why athletes most often use recovery drinks. The main negative effect of glycogen depletion is running out of energy. Whether you are an endurance athlete or working at the office, your body needs energy to sustain physical and mental activity. Glycogen, or its related processes glycogenolysis and glycogen synthesis, is directly linked to stress. As stress increases, glycogen decreases. And as glycogen decreases, stress increases. There are several negative effects of long-term stress , which is something that affects several endurance athletes. Since depleting glycogen stores is a regular process in endurance sports, stress is a constant factor you should be mindful of. The finding of their study was a significant gain in endurance time till exhaustion in the low-glycogen compared to normal glycogen levels. In addition, they found that low-glycogen improved oxidative capacity citrate synthase activity to a larger extent than commencing all exercise sessions with high-glycogen. The findings of Hansen et al. Subsequently, other research groups tested the same hypothesis by using an alternative model with trained subjects [ 12 , 16 ]. Yeo et al. Interestingly, following the 3-wk intervention period, several markers of training adaption were increased. However, min time-trial performance was similar in both the low-glycogen and high-glycogen group. Although speculative, the similar effect in performance suggests that the low-glycogen group showed a greater training adaptation, relative to their level of training intensity. Hulston et al. Moreover, this was accompanied by increases in oxidation of fatty acids, sparing of muscle glycogen, and greater increases in succinate dehydrogenase and 3-hydroxyacyl-CoA dehydrogenase enzyme activity [ 12 ]. However, with regard to performance, the training with low muscle glycogen availability was not more effective than training with high muscle glycogen levels [ 12 ]. Together, low-glycogen availability affects substrate use during exercise by increasing fatty acid oxidation compared to training with normal glycogen levels; this effect is independent of the subject training status. Recently, Cochran et al. Both groups trained on a total of 6 d over a 2-wk period, with a minimum of one day of rest between training days. Furthermore, subjects completed two identical HIIT sessions on each training day, separated by 3 h of recovery. After two weeks of HIIT, mean power output during a kJ time trial increased to a greater extent in the low-glycogen group compared to the high-glycogen group [ 18 ]. A novel aspect of their study was that the subjects performed whole-body exercise for a relatively short period of time 2 weeks , while the study of Hansen et al. A possible explanation for the different outcomes on performance between low-glycogen studies could be differences in the training status of the subjects. Indeed, it has previously been shown that the effectiveness of nutritional interventions is influenced by the subject training status [ 32 ], possibly because trained subjects depend less on carbohydrate utilization because they have greater metabolic flexibility. Another methodological issue is the selected test used to determine performance. In some studies, self-selected intensities were used, which could be influenced by carbohydrate manipulation. Cochran et al. To summarize, although some studies reported that repetitive low-glycogen training leads to improved performance compared with high glycogen [ 17 , 18 ], extrapolating these findings to sports-specific performance should be done with prudence. First, the study of Hansen et al. Second, as suggested by Yeo et al. Lastly, chronic exercise sessions commencing in the low-glycogen state may enhance the risk for overtraining syndrome [ 35 ] which in turn may result in reduced training capacity [ 36 ]. Resistance exercise is typically characterized by short bursts of nearly maximal muscular contractions. When performing resistance exercise, glycogen is crucial to resynthesize the phosphate pool, which provides energy during high intensity muscle contractions [ 37 ]. According to MacDougall et al. This reduction in glycogen content during exercise is determined by the duration, intensity and volume of the performed exercise bout. The largest reductions in glycogen are seen with high repetitions with moderate load training [ 40 ], an effect that mainly occurs in type II fibers [ 39 ]. It has been demonstrated that a reduction of muscle glycogen affects both isokinetic torque [ 29 ] and isoinertial resistance exercise capacity negatively [ 42 ]. However, this effect is not always evident [ 43 ] and is likely to be affected by the protocol used to induce glycogen depletion [ 44 ]. Based on the assumption that pre-exercise glycogen content can influence exercise performance, it seems that the pre-exercise carbohydrate ingestion requires particular attention [ 44 ]. Although it is widely accepted that carbohydrate ingestion before endurance exercise enhances work capacity [ 45 , 46 ], carbohydrate ingestion before resistance exercise has not been studied to the same extent. The importance of carbohydrates for the resistance exercise-type athlete can be substantiated by the idea that glycogen plays a relatively important role in energy metabolism during resistance exercise. For example, it has been shown that pre-resistance exercise carbohydrate ingestion increases the amount of total work [ 47 — 49 ]. In contrast, other reports show no benefit of carbohydrate ingestion on total work capacity [ 50 , 51 ]. To precisely determine the role of glycogen availability for the resistance exercise athlete more training studies that feature a defined area of outcome measures specifically for performance and adaptation are needed. Activity of the exercise-induced peroxisome proliferator-activated γ-receptor co-activator 1α PGC-1α has been proposed to play a key role in the adaptive response with endurance exercise Fig. Enhanced activity of PGC-1α and increased mitochondrial volume improves oxidative capacity through increased fatty acid β -oxidation and mitigating glycogenolysis [ 52 ]. As a result, muscle glycogen can be spared which might delay the onset of muscle fatigue and enhances oxidative exercise performance. PGC-1α is responsible for the activation of mitochondrial transcription factors e. the nuclear respiratory factors NRF-1 and -2 and the mitochondrial transcription factor A Tfam [ 53 ]. Schematic figure representing the regulation of mitochondrial biogenesis by endurance exercise. In addition exercise reduces skeletal muscle glycogen in the contracting muscles which in turn activates the sensing proteins AMPK and p38 MAPK. Both AMPK and p38 MAPK activate and translocate the transcriptional co-activator PGC-1α to the mitochondria and nucleus. The kinases AMPK, p38 MAPK and SIRT 1 then might phosphorylate PGC-1 α and reduce the acetylation of PGC-1 α, which increases its activity. Thus, endurance exercise leads to more PGC-1 α which over time results in mitochondrial biogenesis. Activation of PGC-1α is amongst others regulated by the major up-stream proteins 5' adenosine monophosphate-activated protein kinase AMPK [ 54 ]. Prolonged endurance type exercise requires a large amount of ATP resulting in accumulation of ADP and AMP in the recruited muscle fibers [ 55 ]. This activates AMPK with the purpose to restore cellular energy homeostasis [ 56 , 57 ]. The rise of ADP and AMP during prolonged endurance type exercise results in the phosphorylation of AMPK at Thr, the active site on the AMPK α subunit [ 58 — 60 ]. Canto and colleagues showed that AMPK action on PGC-1α transcriptional activity is partly regulated by SIRT1, a sirtuin family protein which deacetylates several proteins that contribute to cellular regulation [ 57 ]. Furthermore, it was shown that the acute actions of AMPK on lipid oxidation alter the balance between cellular NAD1 and NADH, which acts as a messenger to activate SIRT1 [ 57 ]. During prolonged endurance type exercise skeletal muscle glycogen reduces, this is sensed by the AMPK β subunit resulting in an activation of AMPK Fig. The AMPK is then also activated through phosphorylation of Thr and this response is likely dependent on the rise of AMP and ADP during exercise. Chan et al suggested that low muscle glycogen availability associates with the phosphorylation of the nuclear P38 mitogen-activated protein kinases p38 MAPK , rather than translocation of p38 MAPK to the nucleus per se [ 61 ]. Accordingly, p38 MAPK particularly phosphorylate the expression of PGC-1α [ 53 , 62 ], whereas AMPK could both phosphorylate and enhance expression of PGC-1α [ 53 , 62 ]. Restricted CHO availability during or after exercise has also been shown to augment phosphorylation of i. activate p38 MAPK [ 63 ] and AMPK [ 15 ]. In another study by Mathai and colleagues it was shown that changes in muscle glycogen correlates with the changes in PGC-1α protein abundance during exercise and recovery [ 64 ]. The majority of the studies show that the PGC-1α mRNA content increased during and directly after exercise and returned to resting levels by 24 h after exercise. However, the studies that measured both PGC-1α mRNA and PGC-1α protein after chronic or acute exercise failed to find increases in both [ 64 ]. Therefore, changes of PGC-1α mRNA content are not necessarily compatible with changes in PGC-1α protein abundance following exercise [ 64 ]. Although these studies suggest that the signalling response to exercise is affected by CHO supply, it remains unclear whether exercise in a glycogen-depleted state can enhance the adaptive signalling response that is required for mitochondrial biogenesis. Thus, AMPK and MAPK 38 play a key role in the transcriptional regulation of mitochondrial biogenesis trough PGC-1α in response to stress. However, the precise role of potential regulators which are responsive to glycogen availability, in the processes of mitochondrial biogenesis, needs to be further elucidated. Another described protein that regulates mitochondrial biogenesis is p53, which appears to be sensitive to changes in glycogen availability [ 65 ]. Previous research has shown that p53 is phosphorylated by AMPK and p38 AMPK [ 66 , 67 ]. Furthermore, p53 is implicated in the stimulation of gene expression of mitochondrial function [ 66 , 67 ]. It has been demonstrated that commencing endurance exercise in a glycogen depleted state upregulates p53 to a larger extent than during exercise in a replenished glycogen state [ 68 ]. However, the influence on PGC-1α mRNA expression is difficult to interpret because the subjects involved were not only on an exercise regime with low glycogen availability, but also on a calorie restricted diet. Accordingly, it remains unknown which potent regulator was responsible for the increase in mitochondrial biogenesis in this study. The precise role of both potential regulators in the processes of mitochondrial biogenesis needs to be further elucidated. Although resistance exercise is mainly recognized as mechanical stimulus for increases in strength and hypertrophy, the aerobic effects following resistance exercise have also been studied. Early investigations have shown that skeletal mitochondrial volume [ 69 ] and oxidative capacity [ 70 ] are unaltered following prolonged resistance exercise. However, it has been recently reported that resistance exercise increases the activity of oxidative enzymes in tissue homogenates [ 19 , 71 ] and respiration in skinned muscle fibers [ 72 ]. Moreover, resistance training augmented oxidative phosphorylation in sedentary older adults [ 73 ] and respiratory capacity and intrinsic function of skeletal muscle mitochondria in young healthy men [ 74 ]. Interestingly, following all exercise modalities, concurrent training induced the most robust improvements in mitochondrial related outcomes and mRNA expression [ 75 ]. Notably, the improvements in mitochondria were independent of age. Therefore, exploring molecular processes regulating the metabolic and oxidative responses with resistance training may lead to a better understanding and eventually to optimized adaptations. Studies examining the effect of low glycogen availability on mitochondrial regulators largely centered on endurance training. However, Camera et al. It appears that the level of glycogen acts as a modulator of processes regulating mitochondrial biogenesis, independent of the nature of exercise stimuli. The supposed mechanism by which p53 is translocated from the nucleus to the mitochondria and subsequently enhances mitochondrial biogenesis is through its interaction with mitochondrial transcription factor A Tfam and also by preventing p53 suppression of PGC-1α activation in the nucleus [ 67 ]. According to the findings of Camera et al. Moreover, the acute metabolic response to resistance exercise can be modulated in a glycogen-dependent manner. However, whether these acute alterations in regulators of mitochondrial biogenesis are sufficient to promote mitochondrial volume and function remains to be elucidated in future long-term training studies. Skeletal muscle mass is maintained by the balance between muscle protein synthesis MPS and muscle protein breakdown MPB rates such that overall net muscle protein balance NPB remains essentially unchanged over the course of the day. The two main potent stimuli for MPS are food ingestion and exercise [ 78 ]. Nutrition, proteins in particular, induces a transient stimulation of MPS and is therefore in itself, i. in the absence of exercise, not sufficient to induce a positive NPB. Likewise, resistance exercise improves NPB, however, the ingestion of protein during the post-exercise recovery period is required to induce a positive NPB [ 79 ]. Thus, both exercise and food ingestion must be deployed in combination in order to create a positive NPB [ 78 ]. To date, only a few studies examined the role of glycogen availability on protein metabolism following endurance exercise [ 30 , 80 , 81 ]. It seems that glycogen availability mediates MPB. An early study from Lemon and Mullin showed that when exercise was performed with reduced glycogen availability nitrogen losses more than doubled, suggesting an increase in MPB and amino acid oxidation [ 80 ]. Subsequently, two other studies [ 30 , 81 ] used the arterial-venous a-v difference method to explore whether exercise in the low glycogen state affects amino acid flux and then estimated NPB. In both studies subjects performed an exercise session in the low-glycogen state, the researchers found a net release of amino acids during exercise indicating an increase in MPB. However, these studies may be methodologically flawed because the a-v balance method only allows for the determination of net amino acid balance. Conclusions about changes in MPS and MPB are therefore of a speculative nature [ 82 ]. A more recent study by Howarth et al. They found that skeletal muscle NPB was lower when exercise was commenced with low glycogen availability compared to the high glycogen group, indicating an increase in MPB and decrease in MPS during exercise. It appears that endurance exercise with reduced muscle glycogen availability negatively influences muscle protein turnover and impairs skeletal muscle repair and recovery from endurance exercise. As described previously, low glycogen could be used as a strategy to augment mitochondrial adaptations to exercise, however, protein ingestion is required to offset MPB and increase MPS. Indeed, recent evidence reported that protein ingestion during or following endurance exercise increases MPS leading to a positive NPB [ 83 , 84 ]. The Akt-mTOR-S6K pathway that controls the process of MPS has been studied extensively [ 85 , 86 ]. However, the effects of glycogen availability with resistance exercise and its effects on these regulatory processes remains to be further scrutinized. Furthermore, work by Churchly et al. did not enhance the activity of genes involved in muscle hypertrophy. Creer et al. mTOR phosphorylation was similar to that of Akt, however, the change was not significant. In a comparable study from Camera et al. Muscle biopsies were taken at rest and 1 and 4 h after the single exercise bout. Although mTOR phosphorylation increased to a higher extent in the normal glycogen group, there were no detectable differences found in MPS suggesting that the small differences in signaling are negligible since MPS was unaffected. However, it should be noted that being in an energy deficit state does not necessarily reflects glycogen levels are low. Hence, the total energy available for the cell to undertake its normal homeostatic processes is less. Summarized, it seems that glycogen availability had no influence on the anabolic effects induced by resistance exercise. However, aforementioned studies on the effects of glycogen availability on resistance exercise-induced anabolic response do not resemble a training volume typically used by resistance-type athletes. Future long-term training studies ~12 weeks are needed to find out whether performing resistance exercise with low glycogen availability leads to divergent skeletal muscle adaptations compared to performing the exercise bouts with replenished glycogen levels. Vice versa, the effect of resistance exercise on endurance performance and VO 2max appears to be marginal [ 95 , 96 ]. However, some studies reported compromised gains in aerobic capacity with concurrent training compared to endurance exercise alone [ 97 , 98 ]. Following the work of Hickson et al. Since a detailed analysis on the interference effect associated with concurrent training is beyond the scope of this review, we refer the reader to expert reviews on the interference effect seen with concurrent training Baar et al. It is thought that endurance exercise results in an activation of AMPK, which inhibits the mTORC1 signaling via tuberous sclerosis protein TSC , and this will eventually suppress MPS resulting in a negative net protein balance. In addition, a higher contractile activity also results in a higher calcium flux, which decreases peptide-chain elongation via activation of eukaryotic elongation factor-2 kinase eEF2k leading to a decreased MPS [ 89 , , ]. However, whether the exercise-induced acute interference between AMPK and mTORC1 entirely explains the blunted strength gains seen with concurrent training is to date obscure. To optimize skeletal muscle adaptations and performance, nutritional strategies for both exercise modes should differ. Indeed, it was recently proposed that, when practicing endurance and resistance exercise on the same day, the endurance session should be performed in the morning in the fasted state, with ample protein ingestion [ ]. While the afternoon resistance exercise session should be conducted only after carbohydrate replenishment with adequate post-exercise protein ingestion [ ]. Furthermore, whether such a nutritional strategy leads to improved performance compared to general recommendations for carbohydrate and protein intake remains elusive. Interestingly, it has been demonstrated that a resistance exercise session subsequently after low-intensity endurance, non-glycogen depleting session could enhance molecular signaling of mitochondrial biogenesis induced by endurance exercise [ ]. Furthermore it is currently unclear whether performing resistance exercise with low-glycogen availability affects the acute anabolic molecular events and whether the effects of these responses possibly result in improved or impaired training adaptation. Furthermore, whether low-glycogen availability during the endurance bout amplifies the oxidative resistance exercise induced response remains to be investigated. It seems that both modes of exercise in a low glycogen state as part of a periodized training regime are interesting in terms of acute expressions of markers involved in substrate utilization and oxidative capacity. However, on the other hand, a sufficient amount of glycogen is essential in order to meet the energetic demands of both endurance and resistance exercise. Most existing information on nutrition and concurrent training adaptation is derived from studies where subjects performed exercise in the fasted state [ — ]. Coffey and colleagues investigated the effects of successive bouts of resistance and endurance exercise performed in different order in close proximity on the early skeletal muscle molecular response [ 76 ]. Although the second exercise bout was performed with different levels of skeletal muscle glycogen content, the subsequent effects on Akt, mTOR and p70 signaling following the second exercise bout remained the same. Prospective long-term concurrent training studies may help to understand the complexity of the impaired adaptation with concurrent training and further determine to what extend the acute signaling antagonism contributes to this. Moreover, the role of nutritional factors in counteracting the interference effect remains to be further elucidated. In this review we summarized the role of glycogen availability with regard to performance and skeletal muscle adaptations for both endurance and resistance exercise. Most of the studies with low-glycogen availability focused on endurance type training. The results of these studies are promising if the acute molecular response truly indicates skeletal muscle adaptations over a prolonged period of time. Unfortunately, these results on low-glycogen availability may be biased because many other variables including training parameters time, intensity, frequency, type, rest between bouts and nutritional factors type, amount, timing, isocaloric versus non-isocaloric placebo varied considerably between the studies and it is therefore difficult to make valid inferences. Furthermore, the majority of the studies with low glycogen availability were of short duration [ 18 ] and showed no changes [ 11 — 17 ], or showed, in some cases decreases in performance [ ]. Nevertheless, reductions in glycogen stores by manipulation of carbohydrate ingestion have shown to enhance the formation of training-induced specific proteins and mitochondrial biogenesis following endurance exercise to a greater extent than in the glycogen replenished state [ 11 — 16 , 18 , 68 ]. For resistance exercise, glycogen availability seemed to have no significant influence on the anabolic effects induced by resistance exercise when MPS was measured with the stable isotope methodology. However, the exercise protocols used in most studies do not resemble a training volume that is typical for resistance-type athletes. Future long-term training studies ~12 weeks are needed to investigate whether performing resistance exercise with low glycogen availability leads to divergent skeletal muscle adaptations compared to performing the exercise bouts with replenished glycogen levels. The role of glycogen availability on skeletal muscle adaptations and performance needs to be further investigated. In particular researchers need to examine glycogen availability when endurance and resistance exercise are conducted concurrently, for example, on the same day or on alternating days during the week. To date, only a few studies have investigated the interactions between nutrient intake and acute response following a concurrent exercise model. We recommend that future research in this field should focus on the following questions:. What is the impact of performing one of the exercise bouts endurance or resistance with low glycogen availability on response of markers of mitochondrial biogenesis of the subsequent endurance or resistance exercise bout? Does the resistance exercise bout need to be conducted with replenished glycogen stores in order to optimize the adaptive response when performed after a bout of endurance exercise? Is nutritional timing within a concurrent exercise model crucial to maximize skeletal muscle adaptations following prolonged concurrent training? To conclude, depletion of muscle glycogen is strongly associated with the degree of fatigue development during endurance exercise. This is mainly caused by reduced glycogen availability which is essential for ATP resynthesis during high-intensity endurance exercise. Furthermore, it is hypothesized that other physiological mechanisms involved in excitation-contraction coupling of skeletal muscle may play a role herein. On the other hand, the low glycogen approach seems promising with regard to the adaptive response following exercise. Therefore, low glycogen training may be useful as part of a well-thought out periodization program. However, further research is needed to further scrutinize the role of low glycogen training in different groups e. highly trained subjects combined with different exercise protocols e. concurrent modalities , to develop a nutritional strategy that has the potential to improve skeletal muscle adaptations and performance with concurrent training. Gibala MJ, Little JP, Macdonald MJ, Hawley JA. Physiological adaptations to low-volume, high-intensity interval training in health and disease. J Physiol. Article CAS Google Scholar. Bebout DE, Hogan MC, Hempleman SC, Wagner PD. Effects of training and immobilization on VO2 and DO2 in dog gastrocnemius muscle in situ. J Appl Physiol CAS Google Scholar. Burelle Y, Hochachka PW. Endurance training induces muscle-specific changes in mitochondrial function in skinned muscle fibers. Article Google Scholar. Charifi N, Kadi F, Feasson L, Costes F, Geyssant A, Denis C. Enhancement of microvessel tortuosity in the vastus lateralis muscle of old men in response to endurance training. Folland JP, Williams AG. The adaptations to strength training: morphological and neurological contributions to increased strength. Sports Med. Cermak NM, Res PT, de Groot LC, Saris WH, van Loon LJ. Protein supplementation augments the adaptive response of skeletal muscle to resistance-type exercise training: a meta-analysis. Am J Clin Nutr. Coffey VG, Moore DR, Burd NA, Rerecich T, Stellingwerff T, Garnham AP, et al. Nutrient provision increases signalling and protein synthesis in human skeletal muscle after repeated sprints. Eur J Appl Physiol. Cermak NM, van Loon LJ. The use of carbohydrates during exercise as an ergogenic aid. Hawley JA, Burke LM. Carbohydrate availability and training adaptation: effects on cell metabolism. Exerc Sport Sci Rev. Bartlett JD, Hawley JA, Morton JP. Carbohydrate availability and exercise training adaptation: Too much of a good thing? Eur J Sport Sci. Cox GR, Clark SA, Cox AJ, Halson SL, Hargreaves M, Hawley JA, et al. Daily training with high carbohydrate availability increases exogenous carbohydrate oxidation during endurance cycling. Hulston CJ, Venables MC, Mann CH, Martin C, Philp A, Baar K, et al. Training with low muscle glycogen enhances fat metabolism in well-trained cyclists. Med Sci Sports Exerc. Morton JP, Croft L, Bartlett JD, Maclaren DP, Reilly T, Evans L, et al. Reduced carbohydrate availability does not modulate training-induced heat shock protein adaptations but does upregulate oxidative enzyme activity in human skeletal muscle. Van Proeyen K, Szlufcik K, Nielens H, Ramaekers M, Hespel P. Beneficial metabolic adaptations due to endurance exercise training in the fasted state. Yeo WK, McGee SL, Carey AL, Paton CD, Garnham AP, Hargreaves M, et al. Acute signalling responses to intense endurance training commenced with low or normal muscle glycogen. Exp Physiol. Yeo WK, Paton CD, Garnham AP, Burke LM, Carey AL, Hawley JA. Skeletal muscle adaptation and performance responses to once a day versus twice every second day endurance training regimens. Hansen AK, Fischer CP, Plomgaard P, Andersen JL, Saltin B, Pedersen BK. Skeletal muscle adaptation: training twice every second day vs. training once daily. Cochran AJ, Myslik F, MacInnis MJ, Percival ME, Bishop D, Tarnopolsky MA, et al. Manipulating carbohydrate availability between twice-daily sessions of high-intensity interval training over two weeks improves time-trial performance. Int J Sport Nutr Exerc Metab. Camera DM, Hawley JA, Coffey VG. Resistance exercise with low glycogen increases p53 phosphorylation and PGC-1alpha mRNA in skeletal muscle. Camera DM, West DW, Burd NA, Phillips SM, Garnham AP, Hawley JA, et al. Low muscle glycogen concentration does not suppress the anabolic response to resistance exercise. Ortenblad N, Nielsen J, Saltin B, Holmberg HC. Ortenblad N, Westerblad H, Nielsen J. Muscle glycogen stores and fatigue. Nielsen J, Holmberg HC, Schroder HD, Saltin B, Ortenblad N. Human skeletal muscle glycogen utilization in exhaustive exercise: role of subcellular localization and fibre type. Nielsen J, Suetta C, Hvid LG, Schroder HD, Aagaard P, Ortenblad N. Subcellular localization-dependent decrements in skeletal muscle glycogen and mitochondria content following short-term disuse in young and old men. Am J Physiol Endocrinol Metab. Duhamel TA, Perco JG, Green HJ. Manipulation of dietary carbohydrates after prolonged effort modifies muscle sarcoplasmic reticulum responses in exercising males. Am J Physiol Regul Integr Comp Physiol. van Loon LJ, Greenhaff PL, Constantin-Teodosiu D, Saris WH, Wagenmakers AJ. The effects of increasing exercise intensity on muscle fuel utilisation in humans. Tsintzas K, Williams C. Human muscle glycogen metabolism during exercise. Effect of carbohydrate supplementation. Bergstrom J, Hultman E. A study of the glycogen metabolism during exercise in man. Scand J Clin Lab Invest. Jacobs I, Kaiser P, Tesch P. Muscle strength and fatigue after selective glycogen depletion in human skeletal muscle fibers. Eur J Appl Physiol Occup Physiol. Blomstrand E, Saltin B. Effect of muscle glycogen on glucose, lactate and amino acid metabolism during exercise and recovery in human subjects. Weltan SM, Bosch AN, Dennis SC, Noakes TD. Preexercise muscle glycogen content affects metabolism during exercise despite maintenance of hyperglycemia. Am J Physiol. Porcelli S, Ramaglia M, Bellistri G, Pavei G, Pugliese L, Montorsi M, et al. Aerobic fitness affects the exercise performance responses to nitrate supplementation. Med Sci Sports Exerc ;47 8 — Stellingwerff T, Boit MK, Res PT, International Association of Athletics F. Nutritional strategies to optimize training and racing in middle-distance athletes. J Sports Sci. Hawley JA. Adaptations of skeletal muscle to prolonged, intense endurance training. Clin Exp Pharmacol Physiol. Petibois C, Cazorla G, Poortmans JR, Deleris G. Biochemical aspects of overtraining in endurance sports : the metabolism alteration process syndrome. Achten J, Halson SL, Moseley L, Rayson MP, Casey A, Jeukendrup AE. Higher dietary carbohydrate content during intensified running training results in better maintenance of performance and mood state. MacDougall JD, Ray S, Sale DG, McCartney N, Lee P, Garner S. Muscle substrate utilization and lactate production. Can J Appl Physiol. Katz A, Broberg S, Sahlin K, Wahren J. Leg glucose uptake during maximal dynamic exercise in humans. Koopman R, Manders RJ, Jonkers RA, Hul GB, Kuipers H, van Loon LJ. Intramyocellular lipid and glycogen content are reduced following resistance exercise in untrained healthy males. Pascoe DD, Costill DL, Fink WJ, Robergs RA, Zachwieja JJ. Glycogen resynthesis in skeletal muscle following resistive exercise. Tesch PA, Colliander EB, Kaiser P. Muscle metabolism during intense, heavy-resistance exercise. Leveritt M, Abernethy PJ. Effects of carbohydrate restriction on strength performance. J Strength Cond Res. Google Scholar. Mitchell JB, DiLauro PC, Pizza FX, Cavender DL. The effect of preexercise carbohydrate status on resistance exercise performance. Int J Sport Nutr. Slater G, Phillips SM. Nutrition guidelines for strength sports: sprinting, weightlifting, throwing events, and bodybuilding. Burke LM, Hawley JA, Wong SH, Jeukendrup AE. Carbohydrates for training and competition. Jeukendrup A. A step towards personalized sports nutrition: carbohydrate intake during exercise. Lambert CP, Flynn MG, Boone Jr JB, Michaud TJ, Rodriguez-Zayas J. Effects of carbohydrate feeding on multiple-bout resistance exercise. Haff G, Schroeder C, Koch A, Kuphal K, Comeau M, Potteiger J. The effects of supplemental carbohydrate ingestion on intermittent isokinetic leg exercise. J Sports Med Phys Fitness. Haff GG, Stone MH, Warren BJ, Keith R, Johnson RL, Nieman DC, et al. The effect of carbohydrate supplementation on multiple sessions and bouts of resistance exercise. Kulik JR, Touchberry CD, Kawamori N, Blumert PA, Crum AJ, Haff GG. Supplemental carbohydrate ingestion does not improve performance of high-intensity resistance exercise. Haff GG, Koch AJ, Potteiger JA, Kuphal KE, Magee LM, Green SB, et al. Carbohydrate supplementation attenuates muscle glycogen loss during acute bouts of resistance exercise. Margolis LM, Pasiakos SM. Optimizing intramuscular adaptations to aerobic exercise: effects of carbohydrate restriction and protein supplementation on mitochondrial biogenesis. Adv Nutr. Jager S, Handschin C, St-Pierre J, Spiegelman BM. AMP-activated protein kinase AMPK action in skeletal muscle via direct phosphorylation of PGC-1alpha. Proc Natl Acad Sci U S A. Psilander N, Frank P, Flockhart M, Sahlin K. Exercise with low glycogen increases PGC-1alpha gene expression in human skeletal muscle. Drake JC, Wilson RJ, Yan Z. Molecular mechanisms for mitochondrial adaptation to exercise training in skeletal muscle. Faseb J. Mounier R, Theret M, Lantier L, Foretz M, Viollet B. Expanding roles for AMPK in skeletal muscle plasticity. Trends Endocrinol Metab. Canto C, Gerhart-Hines Z, Feige JN, Lagouge M, Noriega L, Milne JC, et al. Wackerhage H. Molecular Exercise Physiology: An Introduction. Xiao B, Sanders MJ, Underwood E, Heath R, Mayer FV, Carmena D, et al. Structure of mammalian AMPK and its regulation by ADP. Carling D, Thornton C, Woods A, Sanders MJ. AMP-activated protein kinase: new regulation, new roles? |
Glycogen and Resistance Training | Higher muscle glycogen content allows soccer players to spend more time in moderate- to high-speed running and allows hockey players to skate longer and faster during each shift. Risks of Low Muscle Glycogen Content Athletes with low muscle glycogen content will experience a decrease in exertion capacity as well as an increased risk for overtraining and muscle damage. Due to the high demand for glycogen as energy for exertion, many athletes have some pattern of glycogen depletion. This situation may lead to muscle damage and chronic overtraining. In fact, muscle damage limits the capacity of the muscles to store glycogen, so even while consuming a high-carbohydrate diet, an athlete can have difficulty maintaining adequate glycogen stores if the muscles are damaged. Research indicates a correlation between training and competing with high muscle glycogen content and improved exertion capacity and overall performance. Results suggest that muscle glycogen availability can affect performance during both short-term and more prolonged high-intensity intermittent exercise 1. Additionally, MuscleSound delivers immediate data with post-performance scans that can identify the warning signs of muscle fatigue, muscle damage and overtraining. This post-performance insight allows for the concentrated muscle recovery necessary to optimize consistent future performance and prevent long-term muscle injury. MuscleSound allows users to not only optimize, but also capitalize on, the reliable and regular measurement of muscle glycogen content with their patented scientific methodology, practical technology and cloud-based software. Monosaccharides and disaccharides can also be referred to as sugars. Glucose blood sugar is an important monosaccharide that provides energy for muscle contractions 1. Glucose is stored as a specific polysaccharide in our bodies called glycogen. Many molecules of Glucose are chained together to form glycogen, which is stored in our muscles and liver 1. Glycogen is broken down into individual glucose molecules in muscle cells when needed for energy production. Glycogen is essentially stored carbohydrate, and as we know , carbohydrate as a substrate for endurance exercise is very important. Glycogen is mainly stored in our muscle fibers and liver 1 and is readily available for use during exercise. A few landmark early studies have set the stage for why glycogen is so important. These studies showed that:. These results have been backed up and confirmed by many related studies 4,11, It is clear that glycogen is important, and the amount of glycogen that you have is also important. A normal, healthy 70 kg male eating a high carbohydrate diet might have around g 2, calories of carbohydrate stored as glycogen in their muscles, plus another 90g in the liver 5,6,7. Compare this to about 10g of carbohydrates in the bloodstream 5,6,7 , and you can quickly see why glycogen is vital as a source of carbohydrates during exercise. A high carbohydrate diet is key to maintaining and maximizing glycogen stores 5,6,7. This is pretty straightforward, in order to store carbohydrates, you need to first eat carbohydrates. |
Carbohydrates | Read this article on the Outside app available now on iOS devices for members! It has been known in the scientific community since the late s that the ability to perform endurance exercise is strongly influenced by the amount of pre-exercise glycogen carbohydrate stored in skeletal muscles, with muscle glycogen depletion becoming the decisive factor limiting prolonged exercise at moderate intensities 65 to 75 percent of maximum aerobic power, VO 2 max. Any marathoner who has hit the wall knows this intimately as well. It is also well known that more glycogen in muscles before exercise results in a greater use of glycogen during exercise, and therefore increases the ability to sustain a high intensity e. Research has even shown that fatigue can be delayed with carbohydrate supplementation during exercise. When muscle glycogen is depleted by prolonged exercise, muscles respond to the empty tank by synthesizing and storing more than what was previously present, a process largely controlled by the hormone insulin. Empty a full tank, and you get a refilled larger tank in its place. Imagine if your car, after driving for long enough that it ran out of gas, created a larger gas tank when sitting on the driveway. When it comes to refilling a larger tank and inducing greater mitochondrial enzyme activity, which enhances aerobic metabolism , training twice every second day is superior to training once daily. That was the conclusion of researchers at RMIT University in Victoria, Australia, after 18 endurance-trained, male cyclists and triathletes trained for three weeks. Half of the study participants trained six days per week, alternating days of minutes of cycling at 70 percent of their VO 2 max with days of high-intensity interval training. The other half did both of those workouts on the same day separated by one to two hours of rest every other day. After three weeks of training, muscle glycogen significantly increased in the twice-per-day training group but not in the once-per-day group. Aerobic enzyme activity and the amount of fat used during submaximal exercise also increased more by training twice every second day compared with training once daily. A clever experiment at the Copenhagen Muscle Research Center in Copenhagen, Denmark also found that training one leg twice every second day for ten weeks caused greater muscle glycogen storage and greater endurance measured as time to exhaustion during knee extension exercise at 90 percent of peak power output compared to training the other leg of the same person once daily. Starting workouts with low muscle glycogen increases the transcription of specific genes and proteins involved in training adaptation, making it a promising strategy to enhance glycogen storage, which is a crucial factor for long races marathon, ultramarathon. When that threat exists, your DNA gets busy transcribing genes that ultimately lead to making a bigger glycogen fuel tank to assuage the threat. To prepare your body to store more glycogen for long races, low-glycogen training can be accomplished several ways:. Glycogen-depleted muscles force your muscles to more effectively rely on fat for energy, stimulate your liver to make new glucose from non-carbohydrate sources a process called gluconeogenesis , and stimulate a greater synthesis and storage of glycogen during recovery, all of which are important adaptations to prepare for long races, most notably marathons and ultramarathons. To create the largest muscle glycogen storage possible, you need to deplete muscle glycogen on a regular basis. Since you should never do anything different in the marathon that you have not done in training, you must balance the physiological adaptations with the practical concerns. Gycogen and Resistance Training Todd Astorino, M. and Len Kravitz, Ph. Studies Reviewed: Haff, G. The effect of carbohydrate supplementation on multiple sessions and bouts of resistance exercise. Journal of Strength and Conditioning Research, 13, 2 , Leveritt, M. Effects of carbohydrate restriction on strength performance. Journal of Strength and Conditioning Research, 13, 1 , The Role of Glycogen in Aerobic and Resistance Exercise The role of glycogen stored carbohydrate in muscle in aerobic exercise has been clearly shown to be associated with increased work output and duration Haff et al. Carbohydrate is the bodys preferred substrate during endurance exercise due to its more efficient energy yield per liter of oxygen consumed. Previous resistance training research suggests that weight training is associated with a consequential depletion of muscle glycogen stores. For instance, Robergs et al. This article will review two recent articles that further elucidate the role of glycogen in resistance exercise. It is hoped that the personal trainer will gain a better understanding as to the appropriateness of carbohydrate replenishment recommendations for clients engaged in resistance exercise programs. Energy for Resistance Exercise Due to the intense and short-term nature of individual bouts of resistance training, it would seem likely that this activity would be highly dependent upon muscle glycogen for ATP provision. In a study by Tesch et al. Biopsies of muscle samples were obtained from the vastus lateralis before and immediately after exercise. This led the authors to conclude that energy sources in addition to muscle glycogen support heavy resistance training. This suggests that intramuscular lipolysis breakdown of triglycerides may also play a role in energy production during repeated high-intensity exercise. Overall, research suggests that intramuscular glycogen is an important fuel supporting weight training exercise, but not the only substrate. Subjects 5 young men and one woman performed resistance exercise under a control CON condition no strenuous exercise for at least 48 hours prior to testing and after a carbohydrate restricted program EXP. The EXP condition included 60 min of submaximal cycling and four 1 minute bouts of maximal exercise, followed by 48 hours of reduced carbohydrate intake. In comparing the CON to the EXP testing condition, the most observable difference was noted in squat performance, with no significant differences in the knee extension trials. However, there was no difference between the CON and the EXP groups at any of the five contractile speeds of isokinetic knee extensions. In explaining the differing outcomes of the squat sets versus the knee extensions sets to an aerobic and carbohydrate restricted program , the authors summarized previous research that has depicted substrate utilization differences in the type of exercise. |
Recover password | Med Sci Sports. To Iron in architecture and design understand this question, Glycogen storage for endurance athletes did a Strong weight loss pills that combines the Glgcogen of multiple endutance reviewed scientific studies. Indeed, recent evidence reported that protein ingestion during or following endurance exercise increases MPS leading to a positive NPB [ 8384 ]. More content by Brittany. Glycogen is the storage form of glucose and carbohydrates CHO. |
Muscle glycogen and energy production | The graph below shows how Dr. Jeukendreup and his group observed that higher carbohydrate intake was associated with faster finish times at the Triathlon World Championships in Kona. Daily intake of CHO varies depending on the level and duration of activity. An excessive carbohydrate diet without the right amount of exercise would lead to an increase in body fat due to the conversion of CHO to fat. Onywera, V. Food and macronutrient intake of elite Kenyan distance runners. San Millán I, González-Haro C, Hill J. Indirect Assessment of Glycogen Status in Competitive Athletes. Med Sci Sports Exerc. Goodman, MN. Amino acid and protein metabolism. In Exercise, nutrition and energy metabolism,eds. Horton, R. TErtujn, New York: Macmillan. Sherman WM. Metabolism of sugars and physical performance. Am J Clin Nutr. Kjaer M, Kiens B, Hargreaves M, Richter EA. Influence of active muscle mass on glucose homeostasis during exercise in humans. Katz A, Broberg S, Sahlin K, Wahren J. Leg glucose uptake during maximal dynamic exercise in humans. Am J Physiol. Costill DL, Hargreaves M. Carbohydrate nutrition and fatigue. Hermansen L, Hultman E, Saltin B. Muscle glycogen during prolonged severe exercise. Acta Physiol Scand. Coyle EF, Coggan AR, Hemmert MK, Ivy JL. Muscle glycogen utilization during prolonged strenuous exercise when fed carbohydrate. J Appl Physiol. Coyle EF, Hagberg JM, Hurley BF, Martin WH, Ehsani AA, Holloszy JO. Carbohydrate feeding during prolonged strenuous exercise can delay fatigue. Coggan AR, Khort WM, Spina RJ, Bier DM, Holloszy JO. Endurance training decreases plasma glucose turnover and oxidation during moderate intensity in men J Appl Physiol. Coggan AR, Coyle EF. Reversal of fatigue during prolonged exercise by carbohydrate infusion or ingestion. Maughan RJ, Greenhaff PL, Leiper JB, Ball D, Lambert CP, Gleeson M. Diet composition and the performance of high-intensity exercise. J Sports Sci. Sahlin K, Katz A, Broberg S. Tricarboxylic acid cycle intermediates in human muscle during prolonged exercise. McConell G, Snow RJ, Proietto J, Hargreaves M. Muscle metabolism during prolonged exercise in humans: influence of carbohydrate availability. Sherman WM, Wimer GS. Insufficient dietary carbohydrate during training: does it impair athletic performance?. Int J Sport Nutr. While storage capacity is increased in highly trained populations, it appears that at the time of exhaustion, muscle glycogen concentrations are lower in untrained i. Recently, understanding of the underpinning mechanisms responsible for improved exercise capacity with elevated glycogen stores has improved. It has been demonstrated that glycogen loading increases exercise capacity by sparing of the intra-myofibrillar glycogen pool and that the intra-myofibrillar pool in type I muscle fibers is the best predictor of prolonged exercise capacity [ 29 ]. During high-intensity exercise efforts e. As a result of the plethora of research demonstrating the importance of muscle glycogen availability, strategies have been devised on how to optimally stimulate muscle glycogen synthesis in the days leading up to competition, a strategy also known as carbohydrate or glycogen loading [ 1 , 32 ]. To achieve muscle glycogen loading, athletes are recommended to consume a very high carbohydrate diet i. However, it is common for athletes to undertake an exercise session in this time frame, during which some of the stored glycogen will be used. In addition, some sports events e. Indeed, evidence indicates that maintaining high muscle glycogen concentration could be difficult in these circumstances [ 33 ]. More research is required to understand how to better sustain glycogen loading over multiple days, but most likely optimized recovery practices and in-race feeding strategies will represent the most effective ways to maintain adequate carbohydrate availability. In addition to this, more research is required to better understand how, and if it is possible to, affect skeletal muscle glycogen storage localization by diet manipulation, especially when time for carbohydrate ingestion is limited. A greater understanding of whether different types of carbohydrate affect the storage localization within muscle is also warranted. While muscle glycogen depletion is more commonly associated with prolonged strenuous endurance exercise, even short but intense exercise bouts can result in significant reduction of muscle glycogen content [ 30 , 34 , 35 ]. Thus, one could assume that because of this, glycogen loading would be warranted. However, the evidence is not as conclusive, as glycogen loading before shorter duration events does not always translate into performance improvements [ 30 , 36 ]. For instance, Sherman and colleagues showed that for runners undertaking a half-marathon, glycogen loading was of no benefit to performance [ 36 ]. Based on this, it is not currently recommended to perform glycogen loading for events shorter than 90 min [ 32 ]. This is especially pertinent for sports where increased BM could significantly hinder performance by reducing sustainable relative exercise intensity e. However, it is important to discern between functional BM i. Whether liver glycogen stores can also be super compensated remains to be established, as currently there is no clear evidence that this is possible [ 20 ]. Varying carbohydrate intake does indeed change fasting liver glycogen concentrations [ 41 ], and this shows that sufficient carbohydrate intake is required to start an exercise session with normal liver glycogen stores. However, whether glycogen stores could be increased to higher-than-normal levels remains to be established. It is often overlooked that if competition is to be performed in the morning after an overnight fast or any period of prolonged fasting , liver glycogen stores may be compromised. Namely, in the postabsorptive phase e. On the contrary, muscle glycogen stores are typically not affected by an overnight fasting period [ 42 ]. As numerous competitions start in the morning, it is pertinent that the meals after overnight fast are designed so that there is a focus on liver glycogen repletion. While this has not been directly assessed, it could be speculated that providing athletes with a mix of different types of monosaccharides would be beneficial. This suggestion is based on previous observations that combining glucose-based carbohydrates with either fructose or galactose offer benefits on liver glycogen synthesis over glucose-based carbohydrates only [ 43 , 44 , 45 ]. Indeed, a recent study found improved exercise capacity with a breakfast consisting of fructose-glucose-based carbohydrates as compared to glucose-based carbohydrates only [ 46 ]. This would advance practical advice not only from the perspective of optimization of liver glycogen levels, but also from the perspective of preventing consequences of rebound hypoglycemia, which can occur in some athletes when exercise bouts are initiated close to a meal [ 47 ]. Current nutritional guidelines for athletes advise to consume carbohydrates during exercise at different rates and in relation to the duration of exercise bouts, as will be discussed later [ 1 , 2 ]. There are currently two proposed mechanisms of carbohydrate ergogenicity. Firstly, carbohydrates can be sensed in the oral cavity, causing an activation of certain brain regions, leading to stimulation of the central nervous system, as shown by improved performance by carbohydrate mouth rinsing [ 49 , 50 , 51 ]. Secondly, and most importantly, carbohydrates provide an additional fuel source for ATP formation during exercise. Carbohydrate ingestion during exercise maintains stable blood glucose levels over long exercise sessions [ 52 ] and maintains carbohydrate oxidation rates despite declining muscle glycogen stores so that ingested carbohydrates substitute endogenous carbohydrate stores [ 53 , 54 ]. In addition to this, exogenous carbohydrates can spare or even completely suppress liver glycogen breakdown [ 55 , 56 ]. While some studies have found sparing of muscle glycogen with carbohydrate supplementation during exercise [ 57 , 58 ], most of the studies assessing whole muscle glycogen utilization did not see this effect [ 28 ] and a recently published study that evaluated different carbohydrate ingestion rates during cycling exercise did not observe sparing of muscle glycogen in a muscle fiber type-specific manner either [ 59 ]. However, more recent evidence indicating the importance of compartmentalized glycogen metabolism to muscle function opens new avenues for investigating the mechanistic basis of carbohydrate feeding during exercise [ 29 ]. Galactose has typically not been recommended to be ingested during exercise due to a belief that it is not as readily oxidized [ 61 , 62 ]. However, recent evidence demonstrates that at moderate dosages i. It remains to be demonstrated directly, but these data indicate that oxidation of galactose during exercise is not limited when provided as lactose at moderate ingestion rates. Thus, lactose as a source of galactose and glucose can be ingested as an alternative carbohydrate source during exercise, at least in lactose-tolerant individuals. It is believed that at this ingestion rate, the sodium-glucose linked transporters SGLT1 in the small intestine become saturated [ 25 ]. This notion is based on the observations that when fructose, whose absorption from the small intestine utilizes a different transporter i. As a result of this, athletes are recommended to ingest mixtures of glucose- and fructose-based carbohydrates when training or competition is longer than 2. Most studies investigating combined ingestion of glucose-based carbohydrates and fructose utilized a glucose:fructose ratio, and this has since become a standard recommendation [ 60 ]. However, a closer examination of the literature reveals that a ratio closer to unity i. Thus, it could be recommended that composite glucose- and fructose-based carbohydrates in a ratio close to unity are ingested irrespective of exercise duration. The suggestion that glucose-fructose mixtures be recommended over single transportable carbohydrates even when exercise duration lies within 1—2. Most studies to date investigating exogenous carbohydrate oxidation rates have been performed on moderately to highly trained athletes, but not in elite athletes whose absolute energy demands can be vastly higher. However, endogenous carbohydrate oxidation was not further spared by the higher carbohydrate dose, and thus whether there would be an additional performance benefit requires clarification. Current evidence indicates that glucose delivery to the active tissue i. Bypassing intestinal absorption by infusing glucose and maintaining glycaemia did not result in higher exogenous glucose oxidation rates [ 74 ], whereas infusing glucose to maintain hyperglycemia i. This occurred without a reduction in muscle glycogen use, but rather with suppression of fat oxidation rates. As oral ingestion of very large amounts of glucose i. Yet, evidence for recommending such high ingestion rates i. It could be that athletes whose energy turnover rates during exercise are very high and thus have a high glucose flux to muscle due to increased blood flow proportional to absolute exercise intensity [ 83 ] could benefit from carbohydrate ingestion rates that are higher than currently recommended, but this requires further evaluation. Additionally, it remains to be established what occurs at intensities in heavy and severe exercise intensity domains, as some evidence indicates that despite higher carbohydrate demands, exogenous carbohydrate oxidation rates do not further increase [ 84 ]. Nonetheless, at present, it cannot be recommended to alter the currently recommended maximal carbohydrate ingestion dose during endurance exercise i. There have been many attempts to increase exogenous carbohydrate oxidation rates by co-ingestion of carbohydrates with other nutrients or nutritional supplements, a topic recently reviewed by Baur and Saunders [ 85 ]. There was no effect from co-ingestion with protein [ 86 ], calcium [ 87 ] or sucralose [ 88 ], use of different glucose polymers [ 89 ] or use of drinks with different osmolality [ 90 ], whereas effects of caffeine co-ingestion yielded mixed results [ 91 , 92 ]. More recently, use of hydrogel-forming carbohydrate drinks and gels has been popularized, and while the majority of studies found this strategy not to affect exogenous carbohydrate oxidation rates [ 95 , 96 ], a recent study found that there could be a positive effect when using solely monosaccharide-based carbohydrate solutions i. More research is required to better understand a potential utility of the hydrogel. While a vast majority of the studies discussed in the present article are based on interventions conducted in thermoneutral conditions, athletes are commonly required to exercise in extreme environments e. In recent years, advances have been made in understanding how these environmental stressors affect carbohydrate metabolism during exercise [ 98 ] and more work is expected to be undertaken in the upcoming years. When comparing exercise at the same relative exercise intensity between hypoxia and normoxia, there appear to be no differences in substrate oxidation rates [ ], whereas if the absolute intensity is matched, there is an increase in carbohydrate oxidation rates [ ]. This is met with a reduced ability to oxidize exogenous carbohydrates during exercise at both the same absolute [ ] and relative intensity [ ] It was hypothesized that this is largely explained by reduced peripheral insulin sensitivity because of increased oxidative stress in hypoxia [ ]. Interestingly, altitude acclimation i. Thus, it appears that sufficient pre-exercise carbohydrate intake plays a crucial role in sustaining intense exercise in hypoxia [ ]. Exercise in the heat at the same absolute intensity is accompanied with increased rates of glycogenolysis and thus glycogen utilization in non-heat acclimatized individuals [ , , ]. This effect is somewhat alleviated with heat acclimation [ ], making another argument towards the importance of undertaking heat acclimation before competing in the heat [ ]. Similarly, as with hypoxia, exogenous carbohydrate oxidation rates in non-heat acclimated athletes are reduced under heat stress [ ]. The underlying mechanisms are not yet completely understood. The latter could in turn result in accumulation of glucose within the cell and a reduced glucose gradient between muscle cells and blood [ , ]. Of note is also the fact that under heat stress, athletes are exercising at a higher relative exercise intensity, which drives increased carbohydrate oxidation rates [ 99 , ]. It remains to be explored whether heat acclimation somehow alleviates reductions in exogenous carbohydrate oxidation rates in the heat. From the applied perspective, currently, the most important solution to circumvent this is combining glucose- and fructose-based carbohydrates so higher exogenous carbohydrate oxidation rates can be achieved than those seen with glucose alone [ ]. The main aim of carbohydrate nutrition in the post-competition period is recovery of liver and muscle glycogen stores. This does not necessarily imply that carbohydrate intake needs to be such that repletion of glycogen stores always needs to be rapid given that the next exercise session might not require full glycogen stores e. It is believed that for a full repletion of muscle glycogen stores, 24—36 h [ 21 ] are required, whereas for the complete repletion of liver glycogen, 11—25 h are needed [ ]. Current nutritional guidelines recommend athletes ingest moderate to high glycemic index carbohydrates as soon as possible at the rate of 1. However, a close examination of the literature reveals that these guidelines are perhaps too simplistic, especially for elite athletes. Scrutiny of the evidence for the optimal dosage of carbohydrates to be ingested in the early hours of post-exercise recovery reveals that there is only one study available comparing 1. While it is difficult to compare results between different studies given that different methodological approaches have been used, it appears that there is a good relationship between the dosage and the amount of muscle glycogen resynthesis spanning at least from 0 to 1. Thus, given that there is also a relationship between training status and the capacity to store muscle glycogen [ 28 ], it could be hypothesized that, absorption permitting, higher ingestion rates would be favorable to elite athletes whose relative proportion of muscle mass is higher. More research is required to elucidate if this is the case. In addition to this, an emerging topic within the post-exercise recovery period, with an aim to improve functional capabilities of athletes, is the type of carbohydrates ingested in recovery. Namely, advances have been made on the type of carbohydrates i. While there appears to be no benefit of ingesting multiple types of carbohydrates i. Advancing these data are studies showing that recovery of cycling exercise capacity is greater after ingestion of a combination of glucose-based carbohydrates and fructose as compared to glucose-based carbohydrates only [ , ], likely because of higher carbohydrate availability within both liver and muscle glycogen pools. It has been hypothesized but not established that combining glucose with both galactose and fructose would result in more rapid replenishment of both glycogen pools [ ]. Interestingly, this strategy did not translate into improved cycling performance [ ]. The results of the latter study are thus surprising. However, a close examination of the results offers a potential explanation and opens new research questions. Namely, two studies [ , ] quantified utilization of in-recovery ingested carbohydrates in the subsequent exercise bout and found an increase in its use, indicating an increased carbohydrate availability. However, the increase of carbohydrate oxidation rates in the study assessing subsequent cycling performance was such that by the time the cycling time trial was initiated, glycogen stores within the body were likely the same in both conditions. Thus, more work is required to define the precise scenarios when a functional benefit can be expected; however, there appears to be a uniform observation that in terms of metabolism, ingestion of composite carbohydrates is beneficial. A summary of current knowledge on the effectiveness of different monosaccharide types on repletion of different glycogen depots i. Based on the current evidence, it could be recommended that athletes seeking to recover glycogen stores as quickly as possible consider ingesting carbohydrates from a combination of glucose-based carbohydrates and fructose to optimally stimulate both liver and muscle glycogen resynthesis. The same recommendation cannot currently yet be given for galactose as whilst combined galactose-glucose favorably affects liver glycogen synthesis it is currently unknown how effective it is in the replenishment of muscle glycogen stores. Short-term recovery of muscle and liver glycogen stores after exhaustive exercise using different combinations of monosaccharides. Fructose-glucose carbohydrate mixtures have been demonstrated to be very effective in replenishment of both muscle and liver glycogen stores. On the other hand, while glucose-based carbohydrates cause robust rates of muscle glycogen replenishment, liver glycogen synthesis rates are inferior as compared to a combination of fructose-glucose- and galactose-glucose-based carbohydrates. No data are currently available for muscle glycogen synthesis rates after ingesting a galactose-glucose mixture. It is hypothesized but not established that combining fructose-galactose-glucose-based carbohydrates would be optimal for post-exercise repletion of both glycogen pools. CHO carbohydrate. Training can be described as undertaking structured workouts with an aim to improve or maintain performance over time by manipulating the structure, intensity, duration and frequency of training sessions [ , , ]. As total energy requirements and, consequently, carbohydrate demands are high in endurance-based sports, it is fair to assume that optimization of carbohydrate intake in these sport disciplines plays an important role. Early sports nutrition guidelines [ ] advised athletes to both train and compete with high carbohydrate availability, and this approach dominated until , when Hansen and colleagues observed that a reduction in carbohydrate availability before certain training sessions in untrained individuals could potentially enhance training adaptations [ ]. In this study, leg kicking exercise training was performed in a week-long training study. Each leg was subjected to a different treatment. Muscle biopsy analysis also showed more positive metabolic adaptations hydroxy acyl-CoA dehydrogenase [HAD] and citrate synthase [CS] activity in the leg training with reduced muscle glycogen stores. While very attractive, the strategy was found to be effective in untrained individuals, and more work was required to see if similar findings could be observed in already trained individuals. As a result, this study was a landmark study paving the way for further investigations into whether different approaches to nutrient availability in trained athletes are beneficial based on different goals: training adaptation or competition performance. In addition to carbohydrate availability manipulations to influence training adaptations, the concept of training the gut also needs to be considered to become a part of the training process to potentially improve tolerance to high carbohydrate ingestion rates during exercise especially [ , ], as the prevalence of gastrointestinal issues during exercise is large [ , ]. While the concept of training with high carbohydrate intakes to improve tolerance to ingested carbohydrates seems warranted, it remains to be established whether such practice leads to improved absorption of ingested carbohydrates and by what mechanisms or leads to just improved tolerance. Recent evidence from rats indicates that a combination of a high carbohydrate diet and exercise does not result in an increased number of glucose transporters in the intestines [ ], and it could be thus speculated that improved tolerance can occur independently of improved absorption capacity. Building from the study by Hansen and colleagues, research started to focus on ways to optimize training adaptations and not necessarily optimize performance within these training sessions in trained individuals. Indeed, studies investigating molecular signaling responses after acute bouts of training with low muscle and liver glycogen stores in trained individuals provided promising results [ 10 , ]. The concept is well described elsewhere [ , ]. Using this approach, some studies demonstrated metabolic benefits, such as reduced reliance on carbohydrates during moderate-intensity exercise [ , ]. However, a recent meta-analysis of nine studies investigating long-term benefits of carbohydrate periodization on performance outcomes suggests that this approach does not always enhance performance in the long term over training with high carbohydrate availability [ ]. Perhaps important to understand when interpreting these data is that large training volumes are accompanied by substantial energy turnover. Even if a training session is initiated with adequate muscle glycogen stores, they will be markedly reduced by the end of it [ 28 ], creating a suitable environment for activation of crucial molecular signaling pathways thought to be responsible for positive adaptations [ ]. One of the fundamental principles of endurance training is achieving sufficient training volume [ , ]. For instance, elite cyclists are reported to cover more than 30, km on the bike in a single year [ ]. Large training volumes are reported in other endurance sports as well [ ]. This provides support for the notion that accumulation of sufficient training volume is of paramount importance among elite endurance athletes. Training with high carbohydrate availability i. Thus, training with low carbohydrate availability should likely be at best viewed as a more time efficient way to train [ , ] rather than the optimal way. Thus, manipulating carbohydrate availability before and during training sessions could affect molecular responses after exercise bouts. However, focusing solely on activation of pathways such as AMPK could be too reductionist, as it does not account for the recovery that is required after such a session, as, for instance, it is well known that protein breakdown is increased during such sessions [ , ]. In addition to this, recent evidence indicates that the time between two exercise sessions rather than carbohydrate availability is the important modulator of the training responses after the second exercise bout [ , ]. To circumvent this, attempts have been made to rescue the reduction in training capacity by utilization of ingestion of ergogenic aids. In line with this, carbohydrate and caffeine mouth rinsing have been shown to improve high-intensity exercise performance when conducted under a carbohydrate-restricted state [ ]. Whether training adaptation can be enhanced with this approach has not been studied. More recently, building on previous work [ ], the effects of delayed carbohydrate feeding in a glycogen depleted state i. While performance outcomes were unclear, delayed carbohydrate feeding enabled maintenance of stable blood glucose concentrations without suppressing fat oxidation rates and thus created a favorable metabolic response. Again, whether such an approach leads to longer-term enhancement in training adaptation remains to be seen. More broadly there is a need to further explore the potential benefits of commencing exercise with low carbohydrate availability to maximize both the metabolic and mechanical i. Another popular reason for undertaking training with low carbohydrate availability is the notion that such an approach would lead to increases in fat oxidation rates during competition and spare endogenous carbohydrate stores with a limited storage capacity and by doing so improve performance [ 18 , ]. A recent study indicated that the capacity to utilize fat during exercise in an overnight fasted state is best correlated with CS activity [ ], a marker of mitochondrial content [ ] that is itself well correlated with training volume [ ]. More research is required to better understand if training and diet can be structured so that substrate oxidation rates would be altered in favor of fat oxidation without being part of general improvements seen with training per se, and whether this could lead to improvements in endurance performance. Unfortunately, the prevalence of relative energy deficiency in sport RED-S remains high [ ]. Building on the previous evidence that sufficient carbohydrate intake can ameliorate symptoms of overtraining [ , ], it has recently been proposed that there might be a link between relative RED-S and overtraining and that a common confounding factor is carbohydrate [ 11 ]. Recent data support an important role for dietary carbohydrate, as low carbohydrate, but not low energy availability, affects bone health markers [ ], and deliberately inducing low carbohydrate availability to promote training adaptations and remaining in energy balance by increasing fat intake does not offer any benefits over a combination of energy and carbohydrate deficit—even more, it can impair glycemic regulation [ ]. Whether carbohydrate availability is the crucial part in the development of RED-S remains to be properly elucidated. Collectively, periodizing carbohydrate intake based on the demands of training and especially an upcoming training session currently appears to be the most sensible approach as it 1 allows the execution of the prescribed training program, 2 minimizes the risk of high carbohydrate availability impeding training adaptations and 3 helps minimize the risk for occurrence of RED-S. A framework for carbohydrate periodization using this concept is depicted in Fig. Framework for carbohydrate periodization based on the demands of the upcoming exercise session. Exercise intensity domain selection refers to the highest intensity attained during the exercise session. The exact carbohydrate requirements are to be personalized based on the expected energy demands of each exercise session. CHO carbohydrates, CP critical power, LT1 lactate threshold 1, LT2 lactate threshold 2, MLSS maximal lactate steady state. While provision of exact recommendations for carbohydrate intake before and during exercise forms part of sports nutrition recommendations provided elsewhere [ 1 , 2 ], we believe that interindividual differences in energy and thus carbohydrate requirements are such that optimization of carbohydrate intake should be personalized based on the demands and the goals of the exercise session one is preparing feeding for. For instance, aggressive provision of carbohydrate intake during exercise deemed beneficial among one population [ 73 ] in another population could lead to unwanted increase in muscle glycogen utilization [ 81 ]. In addition to this, even within sports commonly characterized as featuring extreme energy turnover rates, day-to-day differences are such that provision of exact carbohydrate guidelines would be too inaccurate [ 22 , ]. Thus, personalization of carbohydrate intake during exercise is warranted, as described in the next section. A certain level of personalization of energy and carbohydrate intake has been a standard part of nutritional guidelines for athletes for years [ 1 , 2 , ]. Practitioners and athletes have a wide array of tools available that can help them personalize energy and carbohydrate intake. For instance, energy turnover for past training sessions and even energy requirements of the upcoming training sessions can relatively easily be predicted in sports where wearables exist to accurately quantify external work performed i. Assuming fixed exercise efficiency one can then relatively accurately determine energy turnover during exercise. Knowing the relative exercise intensity of a given training session can further advance the understanding of the carbohydrate demands during exercise, as depicted in Fig. As described in Sect. Thus, it is possible for athletes to predict energy turnover rates during exercise and adjust the carbohydrate intake accordingly. In addition to this, the literature describing the physiological demands of a given sporting discipline can also be very insightful. For instance, energy turnover using gold-standard techniques has been assessed in many sporting contexts, including football [ ], cycling [ 22 ] and tennis [ ]. By knowing the energy demands, structure and goals of an upcoming training session, one can devise a suitable carbohydrate feeding strategy. Besides making predictions on total energy turnover during exercise, it is useful to establish the rate of glycogen breakdown, as very high-intensity efforts can substantially reduce muscle glycogen content without very high energy turnover rates [ 34 , ], especially as low glycogen availability can negatively affect performance [ 30 ]. Attempts have been made to find ways to non-invasively and cost-effectively measure muscle glycogen concentrations e. These data can be useful for practitioners to determine the relative i. However, whilst knowledge of exercise demands can help with tailoring, an implicit assumption is that all athletes will respond in a similar manner to an intervention, which may not be the case. In this respect, despite the present limitations in the practical assessment of muscle glycogen in field settings, gaining more readily accessible information on individual athlete physiological responses could still be of value to achieve higher degrees of personalization than those that current guidelines allow. Recently, use of continuous glucose monitoring CGM devices has been popularized among endurance athletes, with an aim of personalizing carbohydrate intake around exercise for optimal performance. Certainly, knowledge of blood glucose profiles has the advantage that specific physiological data are generated from the individual athlete. These devices have a rich history in the field of diabetes treatment, and their utility has clearly been demonstrated [ ]. For a device to be deemed of use and its use recommended to a wider audience, both of the following criteria must be met: 1 the parameter that the device is measuring should have contextual relevance i. While there is no doubt that CGM devices are useful in non-exercise contexts, their utility during exercise per se remains to be clearly established. Indeed, CGM devices appear to have limited validity during exercise [ , ], and this may be due to the complex nature of blood glucose regulation during varying types and intensities of exercise. Blood glucose concentrations are a result of glucose uptake by the tissue and glucose appearance i. While it has been known for a long time that hypoglycemia can associate with task failure [ ], its occurrence does not always precede it [ ]. Therefore, further investigative work is required to establish whether differential blood glucose profiles using validated technology during exercise can be identified and be used to individualize carbohydrate intake during exercise. In addition to tracking glycaemia during exercise, tracking it throughout the day could also be proven useful. A recent study utilizing CGM devices compared daily blood glucose profiles in elite trained athletes with those in a sedentary population and discovered large discrepancies in blood glucose concentrations throughout the day between both groups [ ]. Elite athletes spent more time in hyper- and hypoglycemia as compared to sedentary controls, giving an appearance that glycemic control might be impaired. While periods of hyperglycemia are expected due to post-exercise high carbohydrate intakes, observations of hypoglycemia occurring especially at night during sleep were somewhat surprising. This knowledge can then be used to potentially individualize strategies to counter these episodes of impaired glycemic control in real time. While utilization of CGM devices during exercise to guide carbohydrate intake during exercise cannot be presently advised, athletes could individualize carbohydrate ingestion rates during exercise by establishing their highest exogenous carbohydrate oxidation rates [ 25 ]. To do this, one requires the ability to know carbon isotope enrichments of the ingested carbohydrates and in expired carbon dioxide. For example, advances have been made in methodology to easier quantify stable carbon isotope abundance in expired air [ ], a methodology currently used for quantification of exogenous carbohydrate oxidation rates [ 25 ]. Thus, this approach could be spun off from research and be used in practice as well to identify carbohydrate intake rate and carbohydrate compositions that optimize exogenous carbohydrate oxidation in individual athletes. Finally, most research to date has investigated carbohydrate intake in a healthy male population, and thus current carbohydrate guidelines are founded on this evidence. Despite decades of intense carbohydrate research within the field of sports nutrition, new knowledge continues to be generated with the potential to inform practice. In this article, we have highlighted recent observations that provide a more contemporary understanding of the role of carbohydrate nutrition for athletes. For example, our article suggests a stronger emphasis be placed on scaling carbohydrate intake before competition to the demands of that subsequent activity, with particular attention paid to the effects of concomitant exercise during the preparatory period. At high ingestion rates during exercise i. Furthermore, short-term recovery may be optimized by combining glucose-fructose to target both liver and muscle glycogen synthesis simultaneously. Finally, there has been substantial investigation into the role of commencing selected exercise sessions with reduced carbohydrate availability to provide a beneficial stimulus for training adaptation. The abovementioned suggestions are designed to build on the wealth of knowledge and recommendations already established for athletes. Nonetheless, what this review has also revealed is that gaps in our current understanding of carbohydrate nutrition and metabolism in relation to exercise performance remain. Some remaining research questions arising from the present article are presented in Table 1. Answering these research questions could allow continued advancement and refinement of carbohydrate intake guidelines and, by doing that, further increase the possibility of positively impacting athletic performance. Burke LM, Hawley JA, Wong SHS, Jeukendrup AE. Carbohydrates for training and competition. J Sports Sci. Article Google Scholar. Thomas DT, Erdman KA, Burke LM. Nutrition and athletic performance. Med Sci Sports Exerc. Evolution of food provision to athletes at the summer Olympic Games. Nutr Rev. Accessed 4 Dec Krogh A, Lindhard J. The relative value of fat and carbohydrate as sources of muscular energy. Biochem J. Bergstrom J, Hultman E. A study of glycogen metabolism in man. J Clin Lab Invest. Katz A. A century of exercise physiology: key concepts in regulation of glycogen metabolism in skeletal muscle. Eur J Appl Physiol. Noakes TD. What is the evidence that dietary macronutrient composition influences exercise performance? A narrative review. Article CAS Google Scholar. Burke LM, Hawley JA, Jeukendrup AE, Morton JP, Stellingwerff T, Maughan RJ. Toward a common understanding of diet-exercise strategies to manipulate fuel availability for training and competition preparation in endurance sport. Int J Sport Nutr Exerc Metab. Burke LM, Hawley JA. Bartlett JD, Hawley JA, Morton JP. Carbohydrate availability and exercise training adaptation: too much of a good thing? Eur J Sport Sci. Stellingwerff T, Heikura IA, Meeusen R, Bermon S, Seiler S, Mountjoy ML, et al. Overtraining syndrome OTS and relative energy deficiency in sport RED-S : shared pathways, symptoms and complexities. Sports Med. Parolin ML, Chesley A, Matsos MP, Spriet LL, Jones NL, Heigenhauser GJF. Regulation of skeletal muscle glycogen phosphorylase and PDH during maximal intermittent exercise. Am J Physiol Endocrinol Metab. Stellingwerff T, Spriet LL, Watt MJ, Kimber NE, Hargreaves M, Hawley JA, et al. Decreased PDH activation and glycogenolysis during exercise following fat adaptation with carbohydrate restoration. Am J Physiol-Endocrinol Metab. Romijn JA, Coyle EF, Sidossis LS, Gastaldelli A, Horowitz JF, Endert E, et al. Regulation of endogenous fat and carbohydrate metabolism in relation to exercise intensity and duration. van Loon LJC, Greenhaff PL, Constantin-Teodosiu D, Saris WH, Wagenmakers AJM. The effects of increasing exercise intensity on muscle fuel utilisation in humans. J Physiol. Leckey JJ, Burke LM, Morton JP, Hawley JA. Altering fatty acid availability does not impair prolonged, continuous running to fatigue: evidence for carbohydrate dependence. J Appl Physiol. Hawley JA, Leckey JJ. Carbohydrate dependence during prolonged, intense endurance exercise. Hetlelid KJ, Plews DJ, Herold E, Laursen PB, Seiler S. Rethinking the role of fat oxidation: substrate utilisation during high-intensity interval training in well-trained and recreationally trained runners. BMJ Open Sport Exerc Med. Jeukendrup AE, Wallis GA. Measurement of substrate oxidation during exercise by means of gas exchange measurements. Int J Sports Med Suppl. Gonzalez JT, Fuchs CJ, Betts JA, van Loon LJC. Liver glycogen metabolism during and after prolonged endurance-type exercise. Burke LM, van Loon LJC, Hawley JA. Postexercise muscle glycogen resynthesis in humans. Plasqui G, Rietjens G, Lambriks L, Wouters L, Saris WHM. Sjödin AM, Andersson AB, Högberg JM, Westerterp KR. Energy balance in cross-country skiers: a study using doubly labeled water. Accessed 30 Nov Hawley JA, Schabort EJ, Noakes TD, Dennis SC. Carbohydrate-loading and exercise performance. An update. Accessed 2 Aug Jeukendrup AE, Jentjens R. Oxidation of carbohydrate feedings during prolonged exercise. Accessed 13 Oct Burke LM. Bergström J, Hermansen L, Hultman E, Saltin B. Diet, muscle glycogen and physical performance. Acta Physiol Scand. Areta JL, Hopkins WG. Skeletal muscle glycogen content at rest and during endurance exercise in humans: a meta-analysis. Jensen R, Ørtenblad N, Stausholm MH, Skjærbæk MC, Larsen DN, Hansen M, et al. Heterogeneity in subcellular muscle glycogen utilisation during exercise impacts endurance capacity in men. Vigh-Larsen JF, Ørtenblad N, Spriet LL, Overgaard K, Mohr M. Muscle glycogen metabolism and high-intensity exercise performance: a narrative review. Nielsen J, Cheng AJ, Ørtenblad N, Westerblad H. Hawley JA, Schabort E, Noakes TD, Dennis SC. Carbohydrate-loading and exercise performance an update. McInerney P, Lessard SJ, Burke LM, Coffey VG, lo Giudice SL, Southgate RJ, et al. Failure to repeatedly supercompensate muscle glycogen stores in highly trained men. Accessed 2 May Barnett C, Carey M, Proietto J, Cerin E, Febbraio MA, Jenkins D. Muscle metabolism during sprint exercise in man: Influence of sprint training. J Sci Med Sport. Gollnick PD, Piehl K, Saltin B. Selective glycogen depletion pattern in human muscle fibres after exercise of varying intensity and at varying pedalling rates. Sherman WM, Costill DL, Fink WJ, Miller JM. Effect of exercise-diet manipulation on muscle glycogen and its subsequent utilization during performance. Int J Sports Med. Variation in total body water with muscle glycogen changes in man. Acta Physiol Scandi. Kreitzman SN, Coxon AY, Szaz KF. Glycogen storage: illusions of easy weight loss, excessive weight regain, and distortions in estimates of body composition. Am J Clin Nutr. Weyand PG, Davis JA. Running performance has a structural basis. J Exp Biol. Faria EW, Parker DL, Faria IE. The science of cycling factors affecting performance-part 2. Nilsson LH, Hultman E. Liver glycogen in man—the effect of total starvation or a carbohydrate-poor diet followed by carbohydrate refeeding. Scand J Clin Lab Investig. Iwayama K, Tanabe Y, Tanji F, Ohnishi T, Takahashi H. Diurnal variations in muscle and liver glycogen differ depending on the timing of exercise. J Physiol Sci. Décombaz J, Jentjens R, Ith M, Scheurer E, Buehler T, Jeukendrup AE, et al. Fructose and galactose enhance postexercise human liver glycogen synthesis. Liver and muscle glycogen repletion using 13C magnetic resonance spectroscopy following ingestion of maltodextrin, galactose, protein and amino acids. Br J Nutr. Fuchs CJ, Gonzalez JT, Beelen M, Cermak NM, Smith FE, Thelwall PE, et al. Sucrose ingestion after exhaustive exercise accelerates liver, but not muscle glycogen repletion compared with glucose ingestion in trained athletes. Podlogar T, Cirnski S, Bokal Š, Verdel N, Gonzalez J. Addition of fructose to a carbohydrate-rich breakfast improves cycling endurance capacity in trained cyclists. Jeukendrup AE, Killer SC. The myths surrounding pre-exercise carbohydrate feeding. Ann Nutr Metab. Stellingwerff T, Cox GR. Systematic review: Carbohydrate supplementation on exercise performance or capacity of varying durations. Appl Physiol Nutr Metab Physiologie appliquee, nutrition et metabolisme. Carter JM, Jeukendrup AE, Jones DA. The effect of carbohydrate mouth rinse on 1-h cycle time trial performance. Carter JM, Jeukendrup AE, Mann CH, Jones DA. The effect of glucose infusion on glucose kinetics during a 1-h time trial. Chambers ES, Bridge MW, Jones DA. Carbohydrate sensing in the human mouth: effects on exercise performance and brain activity. Jeukendrup AE, Moseley L, Mainwaring GI, Samuels S, Perry S, Mann CH. Exogenous carbohydrate oxidation during ultraendurance exercise. J Appl Physiol Accessed 18 Nov Coyle EF, Hagberg JM, Hurley BF, Martin WH, Ehsani AA, Holloszy JO. Carbohydrate feeding during prolonged strenuous exercise can delay fatigue. Coyle EF, Coggan AR, Hemmert MK, Ivy JL. Muscle glycogen utilization during prolonged strenuous exercise when fed carbohydrate. Jeukendrup AE, Wagenmakers AJM, Stegen J, Gijsen AP, Brouns F, Saris WH. Carbohydrate ingestion can completely suppress endogenous glucose production during exercise. Gonzalez JT, Fuchs CJ, Smith FE, Thelwall PE, Taylor R, Stevenson EJ, et al. Ingestion of glucose or sucrose prevents liver but not muscle glycogen depletion during prolonged endurance-type exercise in trained cyclists. Tsintzas OK, Williams C, Boobis L, Greenhaff P. Carbohydrate ingestion and glycogen utilization in different muscle fibre types in man. Carbohydrate ingestion and single muscle fiber glycogen metabolism during prolonged running in men. Fell JM, Hearris MA, Ellis DG, Moran JEP, Jevons EFP, Owens DJ, et al. Carbohydrate improves exercise capacity but does not affect subcellular lipid droplet morphology, AMPK and p53 signalling in human skeletal muscle. Jeukendrup AE. Nutrition for endurance sports: marathon, triathlon, and road cycling. Leijssen DP, Saris WH, Jeukendrup AE, Wagenmakers AJ. Oxidation of exogenous [13C]galactose and [13C]glucose during exercise. Burelle Y, Lamoureux M-C, Péronnet F, Massicotte D, Lavoie C. Comparison of exogenous glucose, fructose and galactose oxidation during exercise using 13C-labelling. Accessed 19 Nov Odell OJ, Podlogar T, Wallis GA. Comparable exogenous carbohydrate oxidation from lactose or sucrose during exercise. Accessed 8 Feb Wagenmakers AJ, Brouns F, Saris WH, Halliday D. Oxidation rates of orally ingested carbohydrates during prolonged exercise in men. Jentjens RLPG, Achten J, Jeukdendrup AE. High Oxidation Rates from Combined Carbohydrates Ingested during Exercise. Wallis GA, Rowlands DS, Shaw C, Jentjens RLPG, Jeukendrup AE. Oxidation of combined ingestion of maltodextrins and fructose during exercise. Jentjens RLPG, Venables MC, Jeukendrup AE. Oxidation of exogenous glucose, sucrose, and maltose during prolonged cycling exercise. Fructose-maltodextrin ratio in a carbohydrate-electrolyte solution differentially affects exogenous carbohydrate oxidation rate, gut comfort, and performance. Am J Physiol Gastrointest Liver Physiol. Accessed 22 Jul Rowlands DS, Houltham S, Musa-Veloso K, Brown F, Paulionis L, Bailey D. Fructose-glucose composite carbohydrates and endurance performance: critical review and future perspectives. Saris W, van Erp-Baart M, Brouns F, Westerterp K, Hoor F. Study on food intake and energy expenditure during extreme sustained exercise: the Tour de France. Inte J Sports Med. Podlogar T, Bokal Š, Cirnski S, et al. Urdampilleta A, Arribalzaga S, Viribay A, Castañeda-Babarro A, Seco-Calvo J, Mielgo-Ayuso J. Effects of vs. Viribay A, Arribalzaga S, Mielgo-Ayuso J, Castañeda-Babarro A, Seco-Calvo J, Urdampilleta A. Hawley JA, Bosch AN, Weltan SM, Dennis SC, Noakes TD. Effects of glucose ingestion or glucose infusion on fuel substrate kinetics during prolonged exercise. Glucose kinetics during prolonged exercise in euglycemic and hyperglycemic subjects. Pflugers Arch-Eur J Physiol. Jentjens RLPG, Jeukendrup AE. High rates of exogenous carbohydrate oxidation from a mixture of glucose and fructose ingested during prolonged cycling exercise. Jentjens RLPG, Achten J, Jeukendrup AE. High oxidation rates from combined carbohyrates ingested during exercise. Hearris MA, Pugh JN, Langan-Evans C, Mann SJ, Burke L, Stellingwerff T, et al. Glucose plus fructose ingestion for post-exercise recovery—greater than the sum of its parts? Smith JW, Pascoe DD, Passe DH, Ruby BC, Stewart LK, Baker LB, et al. Curvilinear dose-response relationship of carbohydrate 0— g·h -1 and performance. Wallis GA, Yeo SE, Blannin AK, Jeukendrup AE. Dose-response effects of ingested carbohydrate on exercise metabolism in women. Carbohydrate dose influences liver and muscle glycogen oxidation and performance during prolonged exercise. Physiol Rep. Joyner MJ, Casey DP. Regulation of increased blood flow Hyperemia to muscles during exercise: a hierarchy of competing physiological needs. Physiol Rev. Pirnay F, Crielaard JM, Pallikarakis N, Lacroix M, Mosora F, Krzentowski G, et al. Fate of exogenous glucose during exercise of different intensities in humans. Baur DA, Saunders MJ. Carbohydrate supplementation: a critical review of recent innovations. Rowlands DS, Wadsworth DP. No effect of protein coingestion on exogenous glucose oxidation during exercise. Narang BJ, Wallis GA, Gonzalez JT. The effect of calcium co-ingestion on exogenous glucose oxidation during endurance exercise in healthy men: a pilot study. Stellingwerff T, Godin J-P, Beaumont M, Tavenard A, Grathwohl D, van Bladeren PJ, et al. Effects of pre-exercise sucralose ingestion on carbohydrate oxidation during exercise. com ORIGINAL RESEARCH Int J Sport Nutr Exerc Metab. Rowlands DS, Wallis GA, Shaw C, Jentjens RLPG, Jeukendrup AE. Glucose polymer molecular weight does not affect exogenous carbohydrate oxidation. Rehrer NJ, Wagenmakers AJM, Beckers EJ, Halliday D, Leiper JB, Brouns F, et al. Gastric emptying, absorption, and carbohydrate oxidation during prolonged exercise. Yeo SE, Jentjens RLPG, Wallis GA, Jeukendrup AE. Caffeine increases exogenous carbohydrate oxidation during exercise. Hulston CJ, Jeukendrup AE. Substrate metabolism and exercise performance with caffeine and carbohydrate intake. Pugh JN, Wagenmakers AJM, Doran DA, Fleming SC, Fielding BA, Morton JP, et al. Probiotic supplementation increases carbohydrate metabolism in trained male cyclists: a randomized, double-blind, placebo-controlled crossover trial. Am J Physiol Endo-crinol Metab. Accessed 26 Nov Rowlands DS, Clarke J, Green JG, Shi X. L-Arginine but not L-glutamine likely increases exogenous carbohydrate oxidation during endurance exercise. Mears SA, Worley J, Mason GS, Hulston CJ, James LJ. Addition of sodium alginate and pectin to a carbohydrate-electrolyte solution does not influence substrate oxidation, gastrointestinal comfort, or cycling performance. Appl Physiol Nutr Metab. Barber JFP, Thomas J, Narang B, Hengist A, Betts JA, Wallis GA, et al. Pectin-alginate does not further enhance exogenous carbohydrate oxidation in running. Med Sci Sports Exerc NLM Medline. Rowe JT, King RFGJ, King AJ, Morrison DJ, Preston T, Wilson OJ, et al. Glucose and fructose hydrogel enhances running performance, exogenous carbohydrate oxidation, and gastrointestinal tolerance. Accessed 24 Dec Pasiakos SM. Annual review of nutrition nutritional requirements for sustaining health and performance during exposure to extreme environments. Maunder E, Plews DJ, Merien F, Kilding AE. Stability of heart rate at physiological thresholds between temperate and heat stress environments in endurance-trained males. Int J Sports Physiol Perform. Shearman S, Dwyer D, Skiba P, Townsend N. Modeling intermittent cycling performance in hypoxia using the critical power concept. The effects of environmental hypoxia on substrate utilisation during exercise: a meta-analysis. J Int Soc Sports Nutr. Response: commentary on the effects of hypoxia on energy substrate use during exercise. Young AJ, Berryman CE, Kenefick RW, Derosier AN, Margolis LM, Wilson MA, et al. Altitude acclimatization alleviates the hypoxia-induced suppression of exogenous glucose oxidation during steady-state aerobic exercise. |
Auf Ihre Frage habe ich die Antwort in google.com gefunden
Ich bin endlich, ich tue Abbitte, aber meiner Meinung nach ist es offenbar.