Cells make energy by breaking Glycolysis in cells macromolecules. Cellular respiration is Glycolsis biochemical process that converts "food energy" from cellls chemical bonds of macromolecules into chemical energy in the form of adenosine Cheesy cauliflower gratin ATP.
The Herbal energy support step of this tightly regulated and ib process is Glyxolysis. The word glycolysis originates from the Latin glyco sugar Glycokysis lysis breakdown. Glycolysis Glycolysis in cells two main intracellular functions: Gpycolysis ATP and generating intermediate metabolites Glycolysis in cells vells into other pathways.
The glycolytic Glycolysis in cells converts one hexose a six-carbon carbohydrate such Cheesy cauliflower gratin glucose cels two triose Glycoolysis three-carbon carbohydrate such as pyruvate, to produce a net total of two molecules of ATP four Glycolhsis, two consumed Glycolysos two molecules of Glycoylsis adenine dinucleotide Ln.
In the mids, Louis Pasteur determined that microorganisms cause the breakdown of glucose in Glyycolysis absence of oxygen fermentation.
NiEduard Buchner Glycolysix that fermentation Glycolyssis can still be carried out in cell-free Lean Mass Exercises extracts by xells open Glgcolysis cell Fueling Performance with Balanced Macronutrients collecting the cytoplasm containing the Glycolysix molecules and organelles.
Glycolysis in cells Hyperthyroidism Support, inCheesy cauliflower gratin, Arthur Harden and William Blood sugar control and stress management discovered that the rate of fermentation decreases cellz the addition of Glycolyysis phosphate Cells i Natural remedies for bloating relief that fermentation requires the presence of both a Glycilysis component later identified to contain a number Glyycolysis enzymes and Gycolysis low molecular weight, heat-stable fraction inorganic ions, ATP, ADP and coenzymes like NAD.
Bywith Glhcolysis effort of many individuals, the complete glycolysis pathway was established cells Gustav Embden, Glycklysis Meyerhof, Jakub Glycolysiz Parnas, and others. Now, Glycollysis is Cheesy cauliflower gratin as the EMP pathway. There are two ways that glucose can enter the cell.
A group of integral GLUT glucose transporter proteins shuttles glucose into the cytosol via facilitated diffusion. Members of the GLUT protein family are present in specific tissues throughout the human body.
Alternatively, transmembrane symporter proteins move glucose against its concentration gradient via secondary active transport. The symporter uses electrochemical energy from pumping ions.
Examples are the sodium-glucose-linked transporters in the small intestine, the heart, the brain, and the kidneys. Under aerobic O 2 -rich and anaerobic O 2 -deficient conditions, glycolysis can start once glucose enters the cell cytosol.
There are two main phases of glycolysis. The first phase is an energy-requiring preparatory step that traps glucose in the cell and restructures the six-carbon backbone so that it can be efficiently cleaved. The second phase releases energy and generates pyruvate. Depending on the oxygen level and the presence of mitochondria, pyruvate may have one of two possible fates.
Under aerobic conditions with mitochondria present, pyruvate enters the mitochondria, undergoing the citric acid cycle and the electron transport chain ETC to be oxidized to CO 2H 2 O, and even more ATP.
In contrast, under anaerobic conditions i. Interestingly, yeast and some bacteria under anaerobic conditions can convert pyruvate to ethanol through a process known as alcohol fermentation. Tight control and regulation of enzyme-mediated metabolic pathways, such as glycolysis, is critical for the proper functioning of an organism.
Control is exerted by substrate limitation or enzyme-linked regulation. Substrate limitation occurs when the concentration of the substrates and products in the cell is near equilibrium.
Consequently, the availability of the substrate determines the rate of the reaction. In enzyme-linked regulation, the concentration of the substrates and products is far from equilibrium.
The activity of three enzymes hexokinase, phosphofructokinase, and pyruvate kinase determines the rate of reaction, which controls the flux of the overall pathway.
Copyright © MyJoVE Corporation. All rights reserved. Faculty Resource Center. High Schools. Sign In. EN - English CN - 中文 DE - Deutsch ES - Español KR - 한국어 IT - Italiano FR - Français PT - Português.
Table of content. Full table of contents. English Deutsch Русский Français Español Português Polski Türkçe Italiano עִבְרִית Nederlands 日本語 한국어 中文 العربية हिंदी norsk svenska dansk. Overview Cells make energy by breaking down macromolecules. Elucidation of Glycolysis In the mids, Louis Pasteur determined that microorganisms cause the breakdown of glucose in the absence of oxygen fermentation.
Destiny of Glucose There are two ways that glucose can enter the cell. Fate of Pyruvate Depending on the oxygen level and the presence of mitochondria, pyruvate may have one of two possible fates. Regulation of Glycolysis Tight control and regulation of enzyme-mediated metabolic pathways, such as glycolysis, is critical for the proper functioning of an organism.
Contact Us. Recommend to library. Research JoVE Journal Methods Collections JoVE Encyclopedia of Experiments Archive. Education JoVE Core JoVE Science Education JoVE Lab Manual Faculty Resource Center Faculty Site.
Authors Overview Publishing Process Editorial Board Scope and Policies Peer Review FAQ Submit. Librarians Overview Testimonials Subscriptions Access Resources Library Advisory Board FAQ.
ABOUT JoVE Overview Leadership Blog.
: Glycolysis in cellsGlycolysis | Biology I | Metabolic Engineering 3: — Gymnastics meal planning Glycolysis in cells 6—10 Glycolysis in cells twice per glucose molecule, this leads cell a net production of Glycllysis. They further removed diphosphoglyceraldehyde as a possible intermediate in glycolysis. Supporting Information S3. So the NAD gets reduced to NADH. Models and data were handled with the Systems Biology Toolbox 2 developed by Schmidt and Jirstrand [70] ; the model is exemplary given as. Wrote the paper: MR UR. |
Glycolysis - Definition and Glycolysis Pathway | The NKR-stimulated NK cells, however, were more active in ATP-linked respiration than the unstimulated cells. The maximal respiration can be assessed by the addition of an uncoupler FCCP, which drives the respiratory chain to operate at maximum capacity. NK cells stimulated by anti-CD16 antibody and NKG2D ligand significantly enhanced their maximal respiration, indicating that these NK cells have more spare respiratory capacity to meet higher energy demand upon NKR engagement Figures 1D,F. Because the expansion of NK cells using feeder cells would probably change the metabolic state of NK cells, thus the expanded NK cells may exhibit different metabolic responses from resting primary human NK cells upon NKR simulation. Therefore, we also performed ECAR and OCR analyses on primary NK cells that were freshly isolated from human PBMCs. Because primary NK cells are less active than the expanded NK cells, we stimulated primary NK cells with anti-CD16 antibody alone, or co-engaged three NK cell receptors using MICA, anti-2B4 antibody and ICAM-1 to induce stronger activating signals for optimal cell activation. The NK cells were successfully activated by these treatments, as indicated by their upregulation of CDa and IFN-γ mRNA expression Figures 2A,B. Unlike expanded NK cells, the glycolytic rate of freshly isolated primary NK cells was further enhanced when the ATP production in OXPHOS was inhibited by oligomycin, indicating that the primary NK cells depend on both glycolysis and OXPHOS to provide ATP and had relatively lower glycolytic machinery compared with the expanded NK cells Figure 2C. Similar to the ex vivo expanded NK cells, we found freshly isolated NK cells to also exhibit significantly increased glycolysis, glycolytic capacity, and OXPHOS upon NKR stimulation Figures 2C—F. Figure 2. Freshly isolated primary NK cells upregulate glycolysis and OXPHOS in response to activating NKR stimulation. NK cells were isolated from human PBMCs and stimulated by either anti-CD16 mAb or MICA plus ICAM-1 and anti-2B4 mAb for 4 h. NK cell activation was examined by A surface CDa expression as determined by flow cytometry and B IFN-γ mRNA level as quantified by RT-PCR. C,D ECAR and OCR analyses performed using Seahorse Extracellular Flux Analyzer as described in Figure 1. Real-time changes of ECAR C and OCR D in NK cells without blue and with red stimulation by anti-CD16 mAb upper panel or MICA plus ICAM-1 and anti-2B4 lower panel were measured. E Quantification of glycolysis and glycolytic capacity of unstimulated and stimulated NK cells. F Quantification of basal respiration, ATP-linked respiration and maximal respiration in NK cells with and without NKR stimulation. Samples were compared using One-way ANOVA with Dunnett's multiple comparisons test B and independent Student's t -test E,F. Taken together, these data indicate that glycolysis and OXPHOS are both elevated in ex vivo expanded and freshly isolated NK cells upon NKR activation. We next explored how NKR-induced metabolic changes affect NK cell functions. One of the major effector functions of NK cells is the secretion of cytokines, e. Hence, we examined how cell metabolism affects NK cell production of IFN-γ upon NKR stimulation. We treated NK cells with 2-deoxyglucose 2-DG , which is an analog of glucose that blocks glycolysis. Anti-CD16 antibody and NKG2D ligand engagement successfully induced IFN-γ production in expanded NK cells as examined by flow cytometry analysis Figures 3A—C. However, NKR-induced IFN-γ production was significantly reduced by the inhibition of glycolysis and OXPHOS. ELISA measurements of IFN-γ also showed that the inhibition of either OXPHOS or glycolysis decreased IFN-γ secretion of both expanded Figures 3D,E and freshly isolated NK cells Figures 3F,G upon NKR engagement. These data suggest that both glycolysis and OXPHOS are required for IFN-γ production upon NKR activation of NK cells. Figure 3. Glycolysis and OXPHOS are required for NKR-induced IFN-γ production of both ex vivo expanded and freshly isolated NK cells. A Flow cytometry analysis of IFN-γ production of NK cells. B,C Quantification of the percentage of IFN-γ-expressing NK cells after various stimulations and treatments as indicated. D,E IFN-γ secretion of expanded NK cells after various stimulations and treatments as indicated. Supernatant were collected after stimulation and IFN-γ was detected by ELISA. F,G IFN-γ secretion of freshly isolated primary human NK cells. Culture supernatant were collected and IFN-γ was measured by ELISA. Samples were compared using One-way ANOVA with Dunnett's test. As cytotoxicity is another important effector function of NK cell, we proceeded to explore how cell metabolism affects NK cell cytotoxicity. The chronic myeloid leukemia cell line K, which lacks MHC class I and expresses abundant activating NKR ligands, is an ideal target for the assessment of NK cell cytotoxicity Due to limiting access to freshly isolated primary human NK cells, we mainly focused on ex vivo expanded NK cells in assessing NK cell cytotoxicity. This approach is also consistent with the protocol adapted for cell therapy, i. We first analyzed the effect of glycolysis inhibition on NK cell cytotoxicity. We started with the short-term inhibition of glycolysis by treating NK cells with 2-DG for 4 h. These NK cells were then co-cultured with K cells in the presence of 2-DG at effector to target E:T ratio of 0. We next introduced a longer-term inhibition of glycolysis by incubating NK cells with glucose-free medium overnight. Inhibiting glycolysis by glucose starvation significantly reduced the killing of K cells by NK cells at all E:T ratios examined, with the most drastic decrease observed at E:T ratio of Figures 4A,D. These data indicate that long-term inhibition of glycolysis impaired NK cell cytotoxicity. Figure 4. Glycolysis supports NK cell cytotoxicity against target K cells. A,B Killing of K cells by glycolysis-inhibited NK cells. Ex vivo expanded NK cells were pretreated for 4 h with 2-DG or incubated overnight with glucose-free GF medium to inhibit glycolysis. The pretreated NK cells were then A co-cultured with cell tracer violet-labeled K cells in the presence of glycolysis inhibitors i. Untreated NK cells co-cultured with K cells served as control. Dead K cells were labeled with Fixable Viability Dyes FVD. C Killing of glycolysis-inhibited K cells by NK cells. K cells were left untreated or pretreated with 2-DG or glucose-free medium for 1 h to inhibit glycolysis. These K cells were then washed twice with PBS before co-culturing with NK cells at various E:T ratios for 1 h. Dead K cells were labeled with FVD. D—F Quantification of the percentage of killing of K cells by NK cells as shown in A—C , respectively. Samples were compared using two-way ANOVA with Dunnett's multiple comparisons test. In the above studies, the metabolic inhibitors were present in the co-culture, thereby affecting not only effector but also target cells. To examine if applying metabolic inhibitors to NK cells alone affects NK cell cytotoxicity, we washed the pretreated NK cells twice with PBS and co-cultured them with untreated K cells in culture medium free of glycolysis inhibitors. Similarly, glucose starvation impaired NK cell cytotoxicity at all E:T ratios examined, with the most drastic decrease at E:T ratio of Figures 4B,E. To investigate if inhibition of glycolysis also affects K cells' susceptibility to NK cytotoxicity, we pretreated K cells with 2-DG or glucose-free medium for 1 h before co-culturing them with NK cells. Inhibiting glycolysis in K cells had no impact on their susceptibility to NK cell cytotoxicity Figures 4C,F. Taken together, these data indicate that glycolysis is critical in supporting the cytotoxicity of ex vivo expanded NK cells. Next, we explored the impact of OXPHOS inhibition on NK cell cytotoxicity. Although untreated NK cells were already potent in killing K cells, we were surprised to observe a drastic increase in the killing of K cells at all E:T ratios examined when OXPHOS was inhibited Figures 5A,D. OXPHOS-inhibited NK cells exhibited comparable killing of K cells at E:T ratio of 0. However, when we washed off the metabolic inhibitors before incubating the pretreated NK cells with K cells, OXPHOS inhibition showed little effect on NK cell cytotoxicity, as they exhibited similar percentage of killing as untreated NK cells Figures 5B,E. Figure 5. OXPHOS is not required for NK cell cytotoxicity but inhibition of OXPHOS renders K cells more susceptible to NK cell cytotoxicity. A,B Killing of K cells by OXPHOS-inhibited NK cells. C Killing of OXPHOS-inhibited K cells by NK cells. These K cells were washed twice with PBS before co-culturing with NK cells at various E:T ratios for 1 h. Samples were compared using independent Student's t -test. Our data above indicated that inhibition of OXPHOS had little impact on NK cell cytotoxicity when applied to NK cells alone but increased the killing of K target cells when given in co-culture. Hence, we next examined if the metabolic inhibitors could change target cells' susceptibility to NK cell cytotoxicity. The inhibitors were washed away before co-culturing the pretreated K cells with untreated NK cells. Interestingly, inhibiting OXPHOS rendered K cells more susceptible to NK cell cytotoxicity Figures 5C,F. Therefore, inhibiting OXPHOS enhanced the susceptibility of K cells to NK cell killing without compromising NK cell cytotoxicity, suggesting that the inhibition of OXPHOS in tumor environment might be advantageous for NK cell-based immunotherapy. Granule exocytosis pathway is one of the major pathways mediating NK cell cytotoxicity Activated NK cells and their target cells form immunological synapses, which facilitate the entry of lytic granules that contain perforin and granzymes into the target cells to induce cell apoptosis via caspase-dependent or -independent pathways. This process is called degranulation and is associated with the expression of CDa on NK cell surface Thus, we explored how cell metabolism affects the granule exocytosis pathway. Subsequently, we stimulated these cells with anti-CD16 antibody or NKG2D ligand. In contrast, OXPHOS-inhibited NK cells did not show a significant decrease in CDa expression Figures 6B,E,F. Similarly, the K cell-induced expression of CDa on NK cells was also dramatically reduced by glycolysis inhibition but was not affected by OXPHOS inhibition Figures 6G,H. Figure 6. NK cell degranulation induced by NKR activation is dependent on glycolysis. A—F The effect of glycolysis or OXPHOS inhibition on CDa expression of NK cells induced by anti-CD16 mAb or MICA plus ICAM These NK cells were stimulated with anti-CD16 mAb or MICA plus ICAM-1 for 4 h. The CDa expression of NK cells were examined by flow cytometry A,B. C—F Quantification of the percentage of CDa-expressing NK cells. I Granzyme B secretion of NK cells subjected to various stimulations and treatments as stated above. Granzyme B secreted by NK cells was quantified using ELISA, and results were normalized to untreated NK cells. G,H The effect of inhibition of glycolysis or OXPHOS on CDa expression of NK cells induced by K cells. Untreated and the pretreated NK cells were washed twice with PBS and then co-cultured with cell tracer violet-labeled K cells for 30 min. CDa expression of NK cells were examined by flow cytometry G. H Quantification of the percentage of CDa-expressing NK cells induced by K cells. Samples were compared using one-way ANOVA with Dunnett's test. We also investigated the impact of cell metabolism on granzyme B secretion of NK cells. Our ELISA data indicated that anti-CD16 antibody and NKG2D ligand increased the granzyme B secretion of NK cells Figure 6I. Similar to the results of CDa expression, granzyme B secretion induced by NKR-activation was abrogated by the inhibition of glycolysis but not OXPHOS. Taken together, our data suggest that glycolysis is essential in supporting NK cell degranulation induced by NKR activation, whereas OXPHOS has little impact on this process. Death receptor pathways also contribute to NK cell cytotoxicity. NK cells express death receptor ligands—FasL, TNF, and TNF-related apoptosis-inducing ligand TRAIL , which recognize the corresponding receptors Fas, TNFR, and TRAILR, respectively, on target cells. Upon receptor-ligand interaction, death-inducing signaling complexes are formed to activate caspase-dependent and -independent pathways that eventually induce cell apoptosis When stimulated with K cells, expanded NK cells showed a more drastic increase in FasL expression than the plate-bound anti-CD16 antibody and NKG2D ligand stimulated cells Figures 7G,H. Similarly, the induction of FasL expression by K cells was significantly inhibited by glucose starvation. In contrast, the inhibition of OXPHOS exerted little effect on the NKR-induced FasL expression of NK cells Figures 7B,E—H. Unstimulated ex vivo expanded NK cells constitutively express a substantial amount of TRAIL, and its expression could not be further increased by anti-CD16 and NKG2D ligand stimulation Figure S2. Inhibition of glycolysis and OXPHOS had no impact on TRAIL expression. Figure 7. Glycolysis supports FasL expression on NK cells activated by NKR. A—F The effect of glycolysis or OXPHOS inhibition on FasL expression of NK cells induced by anti-CD16 mAb or MICA plus ICAM Untreated and the pretreated NK cells were stimulated with anti-CD16 mAb or MICA plus ICAM-1 for 4 h. FasL expression of NK cells were examined by flow cytometry A,B. C—F Quantification of the percentage of FasL-expressing NK cells. G,H The effect of inhibition of glycolysis or OXPHOS on K cell-induced FasL expression of NK cells. NK cells were pretreated to inhibit glycolysis or OXPHOS as stated above. Untreated and the pretreated NK cells were washed twice with PBS and then co-cultured with cell tracer violet-labeled K for 30 min. FasL expression of NK cells was examined by flow cytometry G. H Quantification of the percentage of FasL-expressing NK cells induced by K cells. Together, these results indicate that the cytotoxicity of expanded NK cells induced by NKR stimulation is mainly through CDa expression, granzyme B secretion, and FasL expression, and are highly dependent on glycolysis. Cellular metabolism plays a critical role in shaping immune responses by controlling immune cell differentiation and function. Resting immune cells e. However, pro-inflammatory and effector immune cells e. Recent studies found that resting NK cells have a low basal metabolic rate. However, under long-term cytokine stimulation, mouse or human NK cells upregulate glycolysis and OXPHOS, which are required for their effector functions 12 , In this current study, we investigated the role of metabolism in NKR-induced NK cell activation, which reflects the situation when NK cells are activated by target cells. We found that NKR stimulation elevates glycolysis and OXPHOS in human NK cells. We further demonstrated that IFN-γ production of NK cells induced by NKR stimulation requires both glycolysis and OXPHOS. More importantly, glycolysis is critical for supporting NK cell degranulation and FasL expression and their killing of target cells. Interestingly, inhibition of OXPHOS in the tumor microenvironment might be advantageous for the elimination of tumor cells by NK cells, because it does not affect NK cell cytotoxicity but renders tumor cells more susceptible to killing. Cytokines and NKRs are two major pathways that regulate NK cell functions. NK cells distinguish self and non-self targets via their NKR expression on cell surface. CD16 acts as an Fc receptor that induces antibody-dependent cellular cytotoxicity. Upon CD16 and NKG2D engagement, expanded and primary NK cells secrete cytokine IFN-γ Figure 3 and induce cell cytotoxicity by boosting cell degranulation and FasL expression Figures 2 , 6 , 7. Because ex vivo expanded NK cells, in our experiments, were cultured with IL-2 and feeder cells that express mbIL and mbIL for 14 days, they could be pre-activated and might have different metabolic state from the resting primary NK cells. Thus, we examined the metabolic changes in both expanded and primary NK cells activated by CD16 and NKG2D engagement to explore if they had distinct metabolic responses to NKR stimulation. Indeed, the expanded NK cells had low oxygen consumption rate for ATP production and showed limited increase in glycolytic rate when ATP synthesis was blocked in mitochondria, indicating that they probably depended more on glycolysis than OXPHOS in supplying ATP, while both glycolysis and OXPHOS were found to be important for ATP production in primary NK cells Figures 1 , 2. Interestingly, NKR stimulation induced similar metabolic reprogramming in both expanded and primary NK cells, i. However, ULBP1 apparently induced stronger glycolysis than MICA in expanded NK cells, although both ULBP1 and MICA are the ligands for NKG2D Figures 1C,E. One possible reason is that the molar concentration of ULBP1 used in our experiments is higher than that of MICA, because we coated MICA and ULBP1 on plates with the same mass concentration, but the molecular weight of ULBP1 is smaller than MICA. Besides, the binding of MICA and ULBP1 to the plastic plate might be different, which could also affect the responses of NK cells to these ligands. Previous research reported that mouse NK cells activated by anti-NK1. Therefore, our results indicate that human and mouse NK cells might have different metabolic requirements upon NKR activation. Similar to our findings in NKR-activated NK cells, long-term stimulation of NK cells by cytokines IL-2, IL, and IL showed increased effector functions, which were accompanied by the upregulation of glycolysis and OXPHOS and the increase of nutrient transporters and mitochondrial mass 12 , Collectively, these results indicate both NKR and cytokine activation of human NK cells induce metabolic reprogramming in these cells. We also investigated how metabolic reprogramming contributed to human NK cell effector functions, namely cytokine secretion and cytotoxicity. Previous studies showed that OXPHOS was required for cytokine-induced IFN-γ and granzyme B production by human primary NK cells, and glycolysis was required for the IFN-γ production by primary CD56 bright NK cells in response to IL and IL stimulation Similarly, our data suggested that both expanded and primary NK cells require glycolysis and OXPHOS to support their production and secretion of IFN-γ upon their activation by NKR. Thus, it is possible that, when glycolysis increases, more GAPDH enzymes are released from IFN-γ mRNA and resulting in the increase in IFN-γ translation. Another possible mechanism by which glycolysis supports IFN-γ production might be through affecting histone acetylation of Ifng , as increased glycolysis produces more acetyl-CoA, which elevates histone acetylation of lysine 9 residue H3K9Ac at Ifng loci, thus increasing IFN-γ production Our data showed that glycolysis but not OXPHOS is critical for expanded NK cells to eliminate the target K cells. NK cells induced rapid clearance of K cells as they eliminated most of the target cells within 1. This rapid cytotoxicity is mainly through the granule exocytosis and FasL pathways. Recent studies emphasize the prerequisite of glycolysis in cytotoxicity and CDa expression of educated NK cells 22 , Similarly, our data indicated that the NKR-activated NK cells also require glycolysis for NK cell cytotoxicity, mainly through supporting NK cell degranulation, granzyme B secretion, and FasL expression. It is possible that the rapid killing of target cells by NK cells requires fast metabolic mobilization, so NK cells are more reliant on glycolysis, which could be more rapidly activated than OXPHOS, for their cytotoxicity. Interestingly, inhibiting OXPHOS in K cells made them more susceptible to NK cell killing but did not affect NK cell cytotoxicity. This is in line with a recent study showing that tyrosine kinase inhibitor-resistant chronic myeloid leukemia cells upregulate OXPHOS for their survival, and the combinational use of a tyrosine kinase inhibitor, imatinib with an OXPHOS inhibiting antibiotics, tigecycline can selectively kill chronic myeloid leukemia stem cells Thus, the differentiated responses of NK and K cells toward OXPHOS inhibition make it a promising NK cell-based tumor treatment strategy in the clinic. Another study also reveals the ability of oligomycin to sensitize leukemia cells to tyrosine kinase inhibitor treatment. They demonstrated that the effect is partially through increasing the production of superoxide to induce cell apoptosis Besides, the DNA damage caused by reactive oxygen species was shown to promote the expression of stress-induced activating ligands on multiple myeloma cells Thus, it is possible that the inhibition of OXPHOS in K cells enhances their expression of activating ligands and elevates the superoxide production to increase K cells' susceptibility to NK cell killing. In conclusion, our study reveals important metabolic requirements for NKR-activated human NK cells. Our data suggest that glycolysis and OXPHOS are both increased upon NKR activation of human NK cells. However, glycolysis is more important than OXPHOS for the effector functions of expanded NK cells. In addition, inhibition of OXPHOS in the tumor environment might be advantageous for NK cell-based immunotherapy. The raw data supporting the conclusions of this article will be made available by the authors, without undue reservation, to any qualified researcher. The studies involving human participants were reviewed and approved by the Institutional Review Board of National University of Singapore ZW, SX, and K-PL designed the work and wrote the manuscript. ZW and DG conducted the experiments. SW and LC provided critical reagents. The authors declare that the research was conducted in the absence of any commercial or financial relationships that could be construed as a potential conflict of interest. We thank Yuhan Huang for technical support and the members of our lab for insightful discussions. This work was funded by Singapore Agency for Science Technology and Research. Smyth MJ, Hayakawa Y, Takeda K, Yagita H. New aspects of natural-killer-cell surveillance and therapy of cancer. Nat Rev Cancer. doi: PubMed Abstract CrossRef Full Text Google Scholar. Trinchieri G. Biology of natural killer cells. Adv Immunol. Fogler WE, Volker K, McCormick KL, Watanabe M, Ortaldo JR, Wiltrout RH. J Immunol. PubMed Abstract Google Scholar. Henkart PA. Integrations of the ordinary differential equations were performed with the CVODE from SUNDIALS [71]. The algorithm SSm [72] was used for stochastic global optimization of the parameters and experiment settings using a least squares objective function, which considers the constraints given in the supporting information 2. A bootstrap method [73] , [74] was used for assessment of the parameter confidence intervals with a total of runs. All simulations were carried out on a Linux-based system. As already described by Rehberg et al. Subsequent analytics were applied to at least three individual wells per time point. For the perturbation experiments, cells were grown to the late exponential growth phase at which a sample was taken as time point zero. After 2 h of limitation, a sample was taken as time point zero of the glucose pulse experiment where PBS was removed and 4 mL of fresh GMEM-Z added. The applied analytics are in detail described in [75]. In short, after removal of the supernatant, cells were washed three times with PBS and treated 30 min with trypsin 2. Cells were harvested using a cell scraper. A Vi-Cell TM XR Cell Viability Analyzer Beckman Coulter was used for cell counting and measurement of the diameter distribution. Cell number and cell diameter distribution were used to determine the cell volume. GLC x concentrations in the supernatant were quantified as described by Genzel and Reichl [76] using a Bioprofile plus analyzer Nova Biomedical, relative standard deviation of the method 1. For the measurement of intracellular metabolites, sample preparation was performed as described in detail by Ritter et al. The medium of the wells was discarded and the cell layer was washed with a 0. Quantification of the intracellular metabolites was performed by anion exchange chromatography BioLC system, Dionex in combination with mass spectrometry LC-MS, relative standard deviation of the method 0. The absolute amount of metabolites per well were related to the measured cell volume at respective times of cultivation. To reduce the error, regression analysis was used to interpolate the measured cell volume. The limit of quantification was related to the simulated cell volume. Sensitivity analysis of initial conditions and model parameters. Adjusting the segregated growth model established previously [14] to MDCK cell proliferation in 6-well plates using DMEM medium. Cell number A , mean cell diameter B and extracellular glucose concentration C during MDCK cell cultivations in 6-well plates and DMEM medium with 2. Lines represent the respective simulation result based on the modifications described in supporting information 4. Prediction of ribose 5-phosphate and uridyl diphosphate glucose during cultivation of MDCK cells in DMEM with limited extracellular glucose. Lines represent the respective simulation result based on the parameters of Table 1 and experiment-specific parameters of Table 2. Flow of information and link of experimental data. Green background: Coupling of segregated cell growth model and structured model of glycolysis; red background: coupling of adjusted segregated cell growth model, which renders cell growth under limited GLC x concentrations, to the structured model of glycolysis. Adenosine-based nucleotide pools during perturbation experiments. ATP A — C , ADP D — F and AMP G — I concentrations in three independent perturbation experiments with MDCK cells in 6-well plates. Cells, originating from a cultivation experiment, are limited in extracellular nutrients by removal of medium and addition of phosphate buffered saline PBS , shown in the first column Lim1, A , D , G and second column Lim2, B , E , H. After two hours of incubation, PBS was exchanged by fresh medium Pulse, C , F , I. SBML model for yeast glycolysis adapted to simulate a glucose limitation scenario. Segregated cell growth model coupled to the structured model of glycolysis for simulation of Cult1. The model is provided as. Structured model of glycolysis for simulation of Lim1. The model is provided in the SBML format level 2 version 4. Predicting the glycolytic activity during cell growth in DMEM medium. Flow of information and initial conditions for parameter fitting. Nomenclature for parameter of the segregated cell growth model. The authors are grateful to Frank Stefan Heldt for critical remarks on the manuscript and to Yvonne Genzel for helpful discussions. Conceived and designed the experiments: JBR UR. Performed the experiments: JBR. Analyzed the data: MR. Wrote the paper: MR UR. Developed and employed the model: MR. Article Authors Metrics Comments Media Coverage Reader Comments Figures. Abstract Due to its vital importance in the supply of cellular pathways with energy and precursors, glycolysis has been studied for several decades regarding its capacity and regulation. Author Summary Glycolysis generates biomass precursors and energy from sugars and is therefore a key element in the metabolism of mammalian cells. Introduction The primary metabolism of cells is essential for cell growth and maintenance. Results Glycolytic activity under changing growth regimes In three independent experiments, adherent MDCK cells were grown in 6-well plates with the serum-containing medium GMEM-Z, which provides sufficient amounts of extracellular substrates over the chosen cultivation time. Download: PPT. Figure 1. Scheme of glycolysis model B and its link to the segregated cell growth model A established previously [23]. Upper glycolysis. Figure 2. Metabolites pools of glycolysis during adherent MDCK cell cultivation. Table 1. Initial conditions for the structured model comprising metabolic status, growth status and culture conditions for the simulated experiment. Figure 3. Estimated fluxes for energy and precursors production during adherent MDCK cell cultivation. Lower glycolysis. Exchange of glycolytic metabolites through other reactions. Response of glycolysis to perturbation experiments Limitation experiments. Figure 4. The response of intracellular metabolite pools to perturbation experiments. Pulse experiments. Link to pentose phosphate pathway and glycogenesis The implemented G6PDH and UT mediated conversion of G6P are entry points into the PPP and the glycogenesis, respectively. Figure 5. Intracellular metabolite pools of pentose phosphate pathway and glycogenesis during adherent MDCK cell cultivation. Figure 6. The response of metabolite pools of pentose phosphate pathway and glycogenesis to perturbation experiment. Estimations and predictions for the metabolic activity ATP and biomass precursors generation under modulation of the glucose transport activity. Figure 7. Impact of in silico GLUT modulation on A ATP and B pentose phosphate pathway PPP production rates. Prediction of metabolic activity during growth in different medium. Figure 8. Prediction of glycolytic metabolite pools during cultivation of adherent MDCK cells in DMEM with 2. Discussion Model structure We developed a kinetic description of glycolysis that, coupled to a segregated cell growth model, enabled describing and analyzing the experimental data of this study comprising roughly data points by using a single set of parameters for the enzyme kinetics. Model coupling and simulation The derived kinetic description of glycolysis simultaneously integrates data of three independent cell cultivation experiments, two limitation experiments and one pulse experiment and therefore required coupling to a model that takes explicitly into account the progress of the cell through different growth phases during the cultivation experiments Cult1—3 [23]. Table 2. Measured and estimated parameters of adherent MDCK cell glycolysis used to simultaneously capture all experiments of this study with confidence intervals between 0. Glycolytic activity during cell cultivation Over the full course of cultivation cells pass through several growth-phases with varying cell-specific volumes and with glucose uptake rates that both strongly influence the metabolite pool dynamics Fig. Materials and Methods Model and simulation The differential algebraic equations of the glycolytic model were composed of first order rate laws, Michaelis-Menten and Hill kinetics which describe enzyme activity in dependence of metabolite concentrations and allosteric influences. Coupling of glycolysis to a segregated cell growth model. The concentration of decreases over cultivation time with 1 For the simulation of the perturbation experiments we assumed that the glucose uptake depends on a variable capacity for glucose trans-membrane transport as well as on the affinity of GLUT for such that Eq. Kinetics of glycolytic enzyme reactions. Pentose phosphate pathway and glycogenesis. The model of glycolysis was extended at the expense of two additional parameters by R5P and UGLC: 11 12 The ribose-phosphate diphosphokinase and glycogen synthase with activity Eq. Simulation procedure, initial values and parameter settings. Experiments and analytics Cell cultivation. Supporting Information. Figure S1. s TIF. Figure S2. Figure S3. Figure S4. Figure S5. File S1. s XML. Model S1. s TXT. Model S2. Model S3. Supporting Information S1. s DOCX. Supporting Information S2. Constraints for metabolite exchange with the PPP. Supporting Information S3. Detailed description of enzyme kinetics. Supporting Information S4. Supporting Information S5. Supporting Information S6. Acknowledgments The authors are grateful to Frank Stefan Heldt for critical remarks on the manuscript and to Yvonne Genzel for helpful discussions. Author Contributions Conceived and designed the experiments: JBR UR. References 1. Fromm HJ, Zewe V Kinetic studies of yeast hexokinase. J Biol Chem — View Article Google Scholar 2. Avigad G Stimulation of yeast phosphofructokinase activity by fructose 2,6-bisphosphate. Biochem Biophys Res Commun — View Article Google Scholar 3. Reynard AM, Hass LF, Jacobsen DD, Boyer PD The correlation of reaction kinetics and substrate binding with the mechanism of pyruvate kinase. View Article Google Scholar 4. Teusink B, Passarge J, Reijenga CA, Esgalhado E, van der Weijden CC, et al. Testing biochemistry. Eur J Biochem — View Article Google Scholar 5. Lambeth MJ, Kushmerick MJ A computational model for glycogenolysis in skeletal muscle. Ann Biomed Eng — View Article Google Scholar 6. Rapoport TA, Heinrich R, Rapoport SM The regulatory principles of glycolysis in erythrocytes in vivo and in vitro. A minimal comprehensive model describing steady states, quasi-steady states and time-dependent processes. Biochem J — View Article Google Scholar 7. Rizzi M, Theobald U, Querfurth E, Rohrhirsch T, Baltes M, et al. Biotechnol Bioeng — View Article Google Scholar 8. Visser D, van der Heijden R, Mauch K, Reuss M, Heijnen S Tendency modeling: a new approach to obtain simplified kinetic models of metabolism applied to Saccharomyces cerevisiae. Metab Eng 2: — View Article Google Scholar 9. Chassagnole C, Noisommit-Rizzi N, Schmid JW, Mauch K, Reuss M Dynamic modeling of the central carbon metabolism of Escherichia coli. Biotechnology and Bioengineering 53— View Article Google Scholar Holzhütter HG, Jacobasch G, Bisdorff A Mathematical modelling of metabolic pathways affected by an enzyme deficiency. A mathematical model of glycolysis in normal and pyruvate-kinase-deficient red blood cells. Kotte O, Zaugg JB, Heinemann M Bacterial adaptation through distributed sensing of metabolic fluxes. Mol Syst Biol 6: Hynne F, Danø S, Sørensen PG Full-scale model of glycolysis in Saccharomyces cerevisiae. Biophys Chem — Kremling A, Bettenbrock K, Laube B, Jahreis K, Lengeler JW, et al. Application for diauxic growth on glucose and lactose. Metabolic Engineering 3: — König M, Bulik S, Holzhütter H-G Quantifying the contribution of the liver to glucose homeostasis: a detailed kinetic model of human hepatic glucose metabolism. PLoS Comput Biol 8: e Noguchi R, Kubota H, Yugi K, Toyoshima Y, Komori Y, et al. Mol Syst Biol 9: Bar-Even A, Flamholz A, Noor E, Milo R Rethinking glycolysis: on the biochemical logic of metabolic pathways. Nat Chem Biol 8: — Link H, Kochanowski K, Sauer U Systematic identification of allosteric protein-metabolite interactions that control enzyme activity in vivo. Nat Biotechnol — van Eunen K, Bouwman J, Daran-Lapujade P, Postmus J, Canelas AB, et al. FEBS J — Janke R, Genzel Y, Händel N, Wahl A, Reichl U Metabolic adaptation of MDCK cells to different growth conditions: effects on catalytic activities of central metabolic enzymes. Ritter JB, Genzel Y, Reichl U High-performance anion-exchange chromatography using on-line electrolytic eluent generation for the determination of more than 25 intermediates from energy metabolism of mammalian cells in culture. J Chromatogr B — Niklas J, Schräder E, Sandig V, Noll T, Heinzle E Quantitative characterization of metabolism and metabolic shifts during growth of the new human cell line AGE1. HN using time resolved metabolic flux analysis. Bioprocess Biosyst Eng — Rehberg M, Rath A, Ritter JB, Genzel Y, Reichl U Changes in intracellular metabolite pools during growth of adherent MDCK cells in two diffferent media. Appl Mircobiol Biotechnol View Article Google Scholar Rehberg M, Ritter JB, Genzel Y, Flockerzi D, Reichl U The relation between growth phases, cell volume changes and metabolism of adherent cells during cultivation. J Biotechnol View Article Google Scholar Sussman I, Erecinska M, Wilson DF Regulation of Cellular-Energy Metabolism - the Crabtree Effect. Biochimica Et Biophysica Acta — Zhang JZ, Behrooz A, Ismail-Beigi F Regulation of glucose transport by hypoxia. Am J Kidney Dis — Semenza GL HIF-1 mediates the Warburg effect in clear cell renal carcinoma. J Bioenerg Biomembr — Boiteux A, Hess B Design of glycolysis. Philos Trans R Soc Lond B Biol Sci 5— Moreno-Sanchez R, Rodriguez-Enriquez S, Marin-Hernandez A, Saavedra E Energy metabolism in tumor cells. Exp Mol Pathol — Petelenz-Kurdziel E, Kuehn C, Nordlander B, Klein D, Hong K-K, et al. PLoS Comput Biol 9: e Nash RW, McKay BS, Burke JM The response of cultured human retinal pigment epithelium to hypoxia: a comparison to other cell types. Invest Ophthalmol Vis Sci — Hossler P, Mulukutla BC, Hu W-S Systems analysis of N-glycan processing in mammalian cells. PLoS One 2: e van Eunen K, Dool P, Canelas AB, Kiewiet J, Bouwman J, et al. IET SYSTEMS BIOLOGY 4: — Maier K, Hofmann U, Reuss M, Mauch K Dynamics and Control of the Central Carbon Metabolism in Hepatoma Cells. BMC Syst Biol 4: Kashiwaya Y, Sato K, Tsuchiya N, Thomas S, Fell DA, et al. Atkinson DE, Walton GM Adenosine triphosphate conservation in metabolic regulation. Rat liver citrate cleavage enzyme. Reitzer LJ, Wice BM, Kennell D Evidence that glutamine, not sugar, is the major energy source for cultured HeLa cells. Renner ED, Plagemann PG, Bernlohr RW Permeation of glucose by simple and facilitated diffusion by Novikoff rat hepatoma cells in suspension culture and its relationship to glucose metabolism. Oliveira AP, Ludwig C, Picotti P, Kogadeeva M, Aebersold R, et al. Mol Syst Biol 8: Grimbs S, Selbig J, Bulik S, Holzhütter H-G, Steuer R The stability and robustness of metabolic states: identifying stabilizing sites in metabolic networks. Mol Syst Biol 3: Wu G, Haynes TE, Li H, Yan W, Meininger CJ Glutamine metabolism to glucosamine is necessary for glutamine inhibition of endothelial nitric oxide synthesis. Yasmeen D, Laird AJ, Hume DA, Weidemann MJ Activation of 3-O-Methyl-Glucose Transport in Rat Thymus Lymphocytes by Concanavalin-a - Temperature and Calcium-Ion Dependence and Sensitivity to Puromycin but Not to Cycloheximide. Biochimica Et Biophysica Acta 89— Mazurek S, Boschek C, Eigenbrodt E The role of phosphometabolites in cell proliferation, energy metabolism, and tumor therapy. Bonarius HP, Ozemre A, Timmerarends B, Skrabal P, Tramper J, et al. Petch D, Butler M Profile of energy metabolism in a murine hybridoma: glucose and glutamine utilization. J Cell Physiol 71— DeBerardinis RJ, Mancuso A, Daikhin E, Nissim I, Yudkoff M, et al. Proc Natl Acad Sci U S A — Wahl A, Sidorenko Y, Dauner M, Genzel Y, Reichl U Metabolic flux model for an anchorage-dependent MDCK cell line: Characteristic growth phases and minimum substrate consumption flux distribution. Biotechnology and Bioengineering — Moreno-Sanchez R, Rodriguez-Enriquez S, Saavedra E, Marin-Hernandez A, Gallardo-Perez JC The bioenergetics of cancer: is glycolysis the main ATP supplier in all tumor cells? Biofactors — Reijenga KA, Snoep JL, Diderich JA, van Verseveld HW, Westerhoff HV, et al. Biophysical Journal — Hatanaka M Transport of sugars in tumor cell membranes. Biochim Biophys Acta 77— Marín-Hernández A, Rodríguez-Enríquez S, Vital-González PA, Flores-Rodríguez FL, Macías-Silva M, et al. Flux control by an over-expressed but strongly product-inhibited hexokinase. Koebmann BJ, Westerhoff HV, Snoep JL, Solem C, Pedersen MB, et al. Mol Biol Rep 41— Soboll S, Scholz R, Heldt HW Subcellular metabolite concentrations. Dependence of mitochondrial and cytosolic ATP systems on the metabolic state of perfused rat liver. Snoep JL, Mrwebi M, Schuurmans JM, Rohwer JM, de Mattos MJT Control of specific growth rate in Saccharomyces cerevisiae. Microbiology — Journal of Experimental Biology — Rehberg M, Wetzel M, Ritter JB, Reichl U The Regulation of Glutaminolysis and Citric Acid Cycle Activity During Mammalian Cell Cultivation. In: Proc 12th IFAC Symposium on Computer Applications in Biotechnology CAB , Mumbai 48— Rath AG, Rehberg M, Janke R, Genzel Y, Scholz S, et al. AAT cells. Young CD, Lewis AS, Rudolph MC, Ruehle MD, Jackman MR, et al. Plos One 6: e Johnston M, Kim J-H Glucose as a hormone: receptor-mediated glucose sensing in the yeast Saccharomyces cerevisiae. Biochem Soc Trans — Schmidt H, Siems W, Müller M, Dumdey R, Rapoport SM ATP-producing and consuming processes of Ehrlich mouse ascites tumor cells in proliferating and resting phases. Exp Cell Res — Sidorenko Y, Wahl A, Dauner M, Genzel Y, Reichl U Comparison of metabolic flux distributions for MDCK cell growth in glutamine- and pyruvate-containing media. Biotechnology Progress — Eigenbrodt E, Glossmann H Glycolysis - one of the keys to cancer. Trends Pharmacol Sci 1: — Traut TW Physiological concentrations of purines and pyrimidines. Mol Cell Biochem 1— Liebl B, Mückter H, Doklea E, Fichtl B, Forth W Influence of 2,3-dimercaptopropanol and other sulfur compounds on oxophenylarsine-mediated inhibition of glucose uptake in MDCK cells. Analyst — Cruz HJ, Ferreira AS, Freitas CM, Moreira JL, Carrondo MJT Metabolic responses to different glucose and glutamine levels in baby hamster kidney cell culture. Appl Microbiol Biot — Matsushita K, Uchida K, Saigusa S, Ide S, Hashimoto K, et al. J Pediatr Surg — Nielsen LK, Reid S, Greenfield PF Cell cycle model to describe animal cell size variation and lag between cell number and biomass dynamics. Fadda S, Cincotti A, Cao G A novel population balance model to investigate the kinetics of in vitro cell proliferation: part I. Model development. American Journal of Kidney Diseases — Schmidt H, Jirstrand M Systems Biology Toolbox for MATLAB: a computational platform for research in systems biology. Bioinformatics — Computers in Physics — Egea JA, Rodriguez-Fernandez M, Banga JR, Marti R Scatter Search for Chemical and Bio-Process Optimization. Journal of Global Optimization 37 3 — Joshi M, Seidel-Morgenstern A, Kremling A Exploiting the bootstrap method for quantifying parameter confidence intervals in dynamical systems. Metab Eng 8: — Efron B, Tibshirani R Bootstrap methods for standard errors, confidence intervals, and other measures of statistical accuracy. Statistical Science 1: 54— Ritter JB, Wahl AS, Freund S, Genzel Y, Reichl U Metabolic effects of influenza virus infection in cultured animal cells: Intra- and extracellular metabolite profiling. Bmc Systems Biology 4: Genzel Y, Reichl U Methods in biotechnology: animal cell biotechnology: Vaccine production-state of the art and future needs in upstream processing. |
Top bar navigation | Sometimes called the citric acid cycle because it deals with citric acid. The same thing that's in orange juice or lemons. And then from there we proceed to the electron transport chain. And we learned in the first overview video of cellular respiration that this is where the bulk of the ATP is actually produced. Although it uses raw materials that came out of these phases up here. Now what I want to do in this video is just focus on glycolysis. And this is kind of-- it's sometimes a challenging task because you can really get stuck in the weeds. And I'll show you the weeds in a little bit, and the actual mechanism. And it can be very daunting. But what I want to do is simplify it for you so you can have the big take-aways. And then we can appreciate, and then maybe when we look at the weeds of glycolysis we can make a little bit more sense of it. So glycolysis, or really cellular respiration, it starts off with glucose. And glucose, we know its formula. It's C6H12O6. And I could draw its whole structure; it would take a little time. But I'm just going to focus on the carbon backbone. So it is a ring, or can be a ring. But I'm just going to draw it as six carbons in a row. Now there's two kind of important phases of glycolysis that are good to know. One, I call the investment phase. And the investment phase actually uses two ATPs. So you know, the whole purpose of cellular respiration is to generate ATPs, but right from the get-go I actually have to use two ATPs. But I use two ATPs and then I'm essentially going to break up the glucose into two 3-carbon compounds right here that actually also have a phosphate group on them. The phosphate groups are coming from those ATPs. They also have a phosphate group on them and this is often called-- well, there's a lot of names for it. Sometimes it's called PGAL. You really don't have to know this. Or phosphoglyceraldehyde, really challenging my spelling skills right here. That's not that important to know. All you have to know is in this first phase you use two ATPs. That's why I call it the investment phase. If we use a business analogy, investment phase. And then each of these two PGAL molecules can then go into the payoff phase. So in the payoff phase, each of these PGALs turn into pyruvate. Which is another 3-carbon, but it's reconfigured. But the process of it going to pyruvate-- and let me write pyruvate in blue, because this is something that, at least it's good to know the word. And I'll show you the structure in a second. Sometimes it's called pyruvic acid. Same thing. And that's essentially the end product of glycolysis. So you start off with glucose in the investment phase. You end up in this phosphoglyceraldehyde, which essentially you broke up your glucose and you put a phosphate on either end of it. And then those each independently go through the payoff phase. So you end up with two molecules of pyruvate for every molecule of glucose you started off with. Now you're saying, hey, Sal, there was a payoff phase, what was our payoff? Well our payoff, we got, for each-- let me write this down as a payoff phase. This is our payoff phase. And I apologize for the white background. I did it because, the mechanism I'm showing you, I copy-and-pasted it from Wikipedia, and they had a white background so I just ran with the white background for this video. But I, personally at least, like the black background a lot better. But this is the payoff phase right here. And so when we go from the phosphoglyceraldehyde to the pyruvate or the pyruvic acid, we produce two things. Or I guess we could say we produce three things. We produce, each of these PGALs to pyruvates produce two ATPs. So I'm going to produce two ATPs there, I'm going to produce two ATPs there. And then they each produce an NADH. And I'll do it in a darker color. And of course they're not producing the whole molecule in a vacuum. Essentially what they're doing is they're starting with the raw material of an NAD plus-- so they start off with an NAD plus-- and they essentially reduce it by adding a hydrogen. Remember, we learned a couple of videos ago that you could view reduction as a gain in hydrogen. So the NAD gets reduced to NADH. And then later on, these NADHs are used in electron transport chain to actually produce ATPs. So the big take-away here, if I were to write the reaction that we get for glycolysis, is that you start off with a glucose. And you need some NAD plus. And actually, for every mole of glucose, you're going to need two NAD plusses. You're going to need two ATPs. So I'm just writing all the ingredients that we need to start off with. And then you're going to need-- well, let me say, these guys are going to be ADPs before we turn them to ATPs. So I'll write plus four ADPs. And then, after performing glycolysis-- and let me write it here. Let me write also-- sorry that was ADPs. Let me just rewrite that part right there. Four ADPs. And then you maybe need two phosphate groups. Because we're going to need four phosphate groups. Plus four-- I'll just call them, sometimes they're written like that. But maybe I'll write it like this. Four phosphate groups. And then once you perform glycolysis, you have two pyruvates, you have two NADHs. The NAD has been reduced. It gained a hydrogen. OIL RIG. Reduction is gain an electron. But in the biological sense, we think of it gaining the hydrogen. Because hydrogen is very non-electronegative, so you're hogging its electrons. You've gained its electrons. So two NADHs and then plus these two ATPs get used in the investment phase. That's why I kind of wrote them a little separately. So these two get used. So then you're left with two ADPs. And then these guys, essentially, get turned into ATPs. So plus four ATPs. I guess we didn't need four. We only needed a net of two phosphate groups. Because two jump off of here. And then we need a total of two more to get four jumping on there. But the big picture is, you start with a glucose, you end up with two pyruvates. You use up two ATPs. You get four ATPs. So you have a net of two ATPs formed. Let me write that very big. Net, what you get out of glycolysis, is two ATPs. You get two NADHs that can each later be used in the electron transport chain to produce three ATPs. You get two NADHs and you get two pyruvates, which are going to be re-engineered into acetyl-CoAs that are going to be the raw materials for the Krebs cycle. But these are the outputs of glycolysis. So now that we have that big picture, let's actually look at the mechanism. Because this is a little bit more daunting when you see it here. But we'll see the same themes that I just talked about. We're starting with a glucose right there. It is a six chain. It's in a circle, in a ring. One, two, three, four, five, six carbons. I could write it like that, just to make a huge oversimplification. It goes through a few steps. I use an ATP here. So let me do that in a color. Let me do it in orange whenever I use an ATP. I use one ATP there. And just like I told you, they have a slightly different name for it. But this is the phosphoglyceraldehyde right here. They call it glyceraldehyde 3-phosphate. It's the exact same molecule. Overall, glycolysis converts one six-carbon molecule of glucose into two three-carbon molecules of pyruvate. Detailed steps: Energy-requiring phase. Each step is catalyzed by its own specific enzyme, whose name is indicated below the reaction arrow in the diagram below. The diagram shows the steps of glycolysis. The diagram begins with an image of the chemical structure and name of glucose and an arrow pointing towards the chemical structure and name glucosephosphate. Above the arrow there is 1 inside a circle to indicate the first step in the reaction. The arrow has the enzyme hexokinase written below the arrow, and there is a looped arrow showing ATP going in and ADP being released. The next reaction is indicated by a 2 inside a circle to show glucosephosphate with an arrow pointing at the chemical structure and label fructose 6-phosphate. Below the arrow the enzyme phosphoglucoisomerase is written. Step 3 of the reaction is indicated by a 3 inside a circle and has an arrow pointing from Fructosephosphate towards the chemical structure and label fructose 1,6-bisphosphate. Above the arrow there is a looped arrow showing ATP going in and ADP being released, and the enzyme phosphofructokinase is written below the arrow. Step 4 of the reaction is indicated by a 4 inside a circle, and shows 2 arrows pointing from fructose 1,6-bisphosphate, and the enzyme fructose bisphosphate aldolase is written between the 2 arrows. Next to the top arrow is the chemical structure and name Dihydroxyacetone phosphate, and next to the bottom arrow is the chemical structure and name Glyceraldehyde 3 phosphate. Step 5 of the reaction is indicated by a 5 inside a circle and shows 2 arrows; one arrow is pointing from Dihydroxyacetone phosphate towards Glyceraldehyde 3-phosphate, and the other arrow is pointing from Glyceraldehyde 3-phosphate towards Dihydroxyacetone phosphate. The enzyme Triose phosphate isomerase is written next to the 2 arrows. Step 1. Step 2. Glucosephosphate is converted into its isomer, fructosephosphate. Step 3. This step is catalyzed by the enzyme phosphofructokinase, which can be regulated to speed up or slow down the glycolysis pathway. Step 4. They are isomers of each other, but only one—glyceraldehydephosphate—can directly continue through the next steps of glycolysis. Step 5. Detailed steps: Energy-releasing phase. In the second half of glycolysis, the three-carbon sugars formed in the first half of the process go through a series of additional transformations, ultimately turning into pyruvate. The reactions shown below happen twice for each glucose molecule since a glucose splits into two three-carbon molecules, both of which will eventually proceed through the pathway. Detailed steps of the second half of glycolysis. All of these reactions will happen twice for one molecule of glucose. Glyceraldehydephosphate is converted into 1,3-bisphosphoglycerate. An inorganic phosphate is also a reactant for this reaction, which is catalyzed by glyceraldehydephosphate dehydrogenase. This step converts an ADP to an ATP. This reaction releases a water molecule. Phosphoenolpyruvate PEP is converted to pyruvate by pyruvate kinase. An ADP is converted to an ATP in this reaction. Image modified from " Glycolysis: Figure 2 ," by OpenStax College, Biology CC BY 3. Step 6. The overall reaction is exergonic, releasing energy that is then used to phosphorylate the molecule, forming 1,3-bisphosphoglycerate. Step 7. Step 8. Step 9. Step You can learn how this works in the videos and articles on pyruvate oxidation , the citric acid cycle , and oxidative phosphorylation. It can't just sit around in the cell, piling up. There are two basic ways of accomplishing this. This process is called fermentation , and you can learn more about it in the fermentation videos. Want to join the conversation? Log in. Sort by: Top Voted. Ahmad Nawaz. Posted 8 years ago. Why the 1st phase are same in aerobic and anaerobic respiration. Downvote Button navigates to signup page. Flag Button navigates to signup page. Show preview Show formatting options Post answer. Yeongseo Lee. This is because oxidation in glycolysis doesn't involve oxygen atoms. It's just movement of hydrogen. So it's behaving in the same way with or without oxygen. when NAD is turned into NADH, G3P 5H loses two electrons and "two" protons. but in the next step, 3-bis not sure how this happens. The other H comes from HPO4 with a 2- charge which eventually turns itself into inorganic phosphate. Comment Button navigates to signup page. Stephen Hutchinson. In the Investment phase, where did the 2 atps come from that were used up? was it taken from somewhere else? if the goal is to produce atp in glycilysis, where do we get atp to begin the process? Nicholas Cook-Rostie. The ATPs originally came from your mother through parental nutrition, while you where developing in the womb. When you are born you will have a stock pile of ATP in your body, which must be replenished to stay alive. The body has many ways to make ATP, which can be seen by looking at the vast amount of metabolic reactions that occur with the body. This is also why we can survive for a long time without any additional consumption of food as the many catabolism pathways in the body that breakdown larger molecules and transfer the energy from the breakdown to ATP. |
Overview of glycolysis | To develop more effective combination therapies altering cancer metabolism, it would be useful to improve our understanding of cancer cell metabolism. ATP competes with AMP for the allosteric effector site on the PFK enzyme. Glossary aerobic respiration process in which organisms convert energy in the presence of oxygen anaerobic process that does not use oxygen glycolysis process of breaking glucose into two three-carbon molecules with the production of ATP and NADH isomerase enzyme that converts a molecule into its isomer pyruvate three-carbon sugar that can be decarboxylated and oxidized to make acetyl CoA, which enters the citric acid cycle under aerobic conditions; the end product of glycolysis. Schmidt H, Siems W, Müller M, Dumdey R, Rapoport SM ATP-producing and consuming processes of Ehrlich mouse ascites tumor cells in proliferating and resting phases. Because we're going to need four phosphate groups. Enolase ENO. is likely to be different too. |
ORIGINAL RESEARCH article | Keppel MP, Saucier N, Mah AY, Vogel TP, Cooper MA. Based on the additional quantification of extracellular metabolite changes and cell number measurements a systematic analysis of basic dynamics of glycolysis for various cultivation conditions is possible. Step 1. Posted 8 months ago. Journal of the History of Biology. Furthermore, Schmidt et al. Arch Biochem Biophys — |
Glycolysis in cells -
Step 3. The third step is the phosphorylation of fructosephosphate, catalyzed by the enzyme phosphofructokinase. A second ATP molecule donates a high-energy phosphate to fructosephosphate, producing fructose-1,6- bi sphosphate.
In this pathway, phosphofructokinase is a rate-limiting enzyme. It is active when the concentration of ADP is high; it is less active when ADP levels are low and the concentration of ATP is high. This is a type of end product inhibition, since ATP is the end product of glucose catabolism.
Step 4. The newly added high-energy phosphates further destabilize fructose-1,6-bisphosphate. The fourth step in glycolysis employs an enzyme, aldolase, to cleave 1,6-bisphosphate into two three-carbon isomers: dihydroxyacetone-phosphate and glyceraldehydephosphate. Step 5. In the fifth step, an isomerase transforms the dihydroxyacetone-phosphate into its isomer, glyceraldehydephosphate.
Thus, the pathway will continue with two molecules of a single isomer. At this point in the pathway, there is a net investment of energy from two ATP molecules in the breakdown of one glucose molecule. Second Half of Glycolysis Energy-Releasing Steps So far, glycolysis has cost the cell two ATP molecules and produced two small, three-carbon sugar molecules.
Both of these molecules will proceed through the second half of the pathway, and sufficient energy will be extracted to pay back the two ATP molecules used as an initial investment and produce a profit for the cell of two additional ATP molecules and two even higher-energy NADH molecules.
Step 6. The sugar is then phosphorylated by the addition of a second phosphate group, producing 1,3-bisphosphoglycerate. Note that the second phosphate group does not require another ATP molecule.
Here again is a potential limiting factor for this pathway. If oxygen is available in the system, the NADH will be oxidized readily, though indirectly, and the high-energy electrons from the hydrogen released in this process will be used to produce ATP.
Step 7. In the seventh step, catalyzed by phosphoglycerate kinase an enzyme named for the reverse reaction , 1,3-bisphosphoglycerate donates a high-energy phosphate to ADP, forming one molecule of ATP. This is an example of substrate-level phosphorylation. A carbonyl group on the 1,3-bisphosphoglycerate is oxidized to a carboxyl group, and 3-phosphoglycerate is formed.
Step 8. In the eighth step, the remaining phosphate group in 3-phosphoglycerate moves from the third carbon to the second carbon, producing 2-phosphoglycerate an isomer of 3-phosphoglycerate.
The enzyme catalyzing this step is a mutase isomerase. Step 9. Enolase catalyzes the ninth step. This enzyme causes 2-phosphoglycerate to lose water from its structure; this is a dehydration reaction, resulting in the formation of a double bond that increases the potential energy in the remaining phosphate bond and produces phosphoenolpyruvate PEP.
Step Many enzymes in enzymatic pathways are named for the reverse reactions, since the enzyme can catalyze both forward and reverse reactions these may have been described initially by the reverse reaction that takes place in vitro, under non-physiological conditions.
Gain a better understanding of the breakdown of glucose by glycolysis by visiting this site to see the process in action. Glycolysis starts with glucose and ends with two pyruvate molecules, a total of four ATP molecules and two molecules of NADH.
Two ATP molecules were used in the first half of the pathway to prepare the six-carbon ring for cleavage, so the cell has a net gain of two ATP molecules and 2 NADH molecules for its use. If the cell cannot catabolize the pyruvate molecules further, it will harvest only two ATP molecules from one molecule of glucose.
Mature mammalian red blood cells are not capable of aerobic respiration —the process in which organisms convert energy in the presence of oxygen—and glycolysis is their sole source of ATP. If glycolysis is interrupted, these cells lose their ability to maintain their sodium-potassium pumps, and eventually, they die.
The last step in glycolysis will not occur if pyruvate kinase, the enzyme that catalyzes the formation of pyruvate, is not available in sufficient quantities.
In this situation, the entire glycolysis pathway will proceed, but only two ATP molecules will be made in the second half. Thus, pyruvate kinase is a rate-limiting enzyme for glycolysis.
Glycolysis is the first pathway used in the breakdown of glucose to extract energy. It was probably one of the earliest metabolic pathways to evolve and is used by nearly all of the organisms on earth. Glycolysis consists of two parts: The first part prepares the six-carbon ring of glucose for cleavage into two three-carbon sugars.
ATP is invested in the process during this half to energize the separation. Two ATP molecules are invested in the first half and four ATP molecules are formed by substrate phosphorylation during the second half.
This produces a net gain of two ATP and two NADH molecules for the cell. We found that glycolytic suppression upregulated mitochondrial function and altered the metabolic profile, in particular the metabolites of the TCA cycle and amino acids.
In addition, glycolytic suppression induced autophagy, thereby increasing the amino acid supply and promoting maintenance of mitochondrial function in glycolysis-suppressed cells.
Our findings suggest that intracellular glycometabolism is reprogrammed to use mitochondrial OXPHOS via autophagy when glycolysis is suppressed. Our results provide insight into appropriate strategies for combination therapy targeting cancer metabolism in multiple types of cancer cells.
To examine the metabolic profiles of cancer cells when the glycolytic pathway was suppressed, we replaced the glucose in the culture medium with galactose or a low concentration of glucose low-glucose ; the conditions were based on our previous research Oxidation of galactose to pyruvate through glycolysis yields no net ATP Here, we investigated intracellular metabolism in PANC-1 human pancreatic cancer cells as a representative model of solid tumor cells.
Replacement of sugar source dramatically decreased the release of lactate, the final product of the glycolysis, confirming that changing the sugar source in the culture medium suppressed glycolysis in PANC-1 cells Fig.
In this glycolysis-suppressed condition, we investigated cell proliferation by carboxyfluorescein diacetate succinimidyl ester CFSE staining. CFSE dye is retained within cells, and the fluorescence intensity is reduced by half in the daughter cells following each cell division.
Fluorescence intensity was higher in cells cultured in galactose or low-glucose than in cells cultured in glucose Fig. The reduced cell proliferation in PANC-1 cells cultured with galactose or low-glucose was quantitatively confirmed by growth curves based on cell numbers Fig.
However, merely suppressing glycolysis barely affected cell survival, as shown by the marginal increase in the percentage of propidium iodide PI -positive cells cultured in galactose or low-glucose Fig.
Taken together, these data indicate that glycolysis-suppressed PANC-1 cells could survive, although their growth was mildly suppressed. Glycolytic suppression dynamically changes the glycometabolism profile of PANC-1 cells.
Data are representative of three independent cell cultures. d Survival rate of the cells in a was evaluated based on PI uptake, as determined by flow cytometry.
e The levels of metabolites extracted from PANC-1 cells cultured in Glc, Gala, or Low-glc medium were measured by CE-TOFMS and normalized against the amount of total cellular protein.
Metabolomic patterns were visualized using z-score plots and heat maps. Data represent two independent cell cultures for each condition. Therefore, we speculated that another energy acquisition pathway helps PANC-1 cells to survive when the glycolytic pathway is inhibited.
To investigate alternative pathways, we used CE-TOFMS to monitor intracellular metabolites related to glycometabolism in glycolysis-suppressed PANC-1 cells. CE-TOFMS-based metabolomics can analyze water-soluble charged metabolites, including the glycolytic products and intermediates of the TCA cycle, as well as amino acids.
The level of lactate was dramatically decreased in cells cultured in galactose or low-glucose, confirming that glycolysis was suppressed. Interestingly, the levels of all intermediates of the TCA cycle were dramatically reduced in PANC-1 cells cultured in galactose or a low-glucose.
On the other hand, the levels of amino acids alanine, arginine, asparagine, glycine, histidine, isoleucine, leucine, lysine, methionine, phenylalanine, proline, serine, threonine, tryptophan, tyrosine, and valine were increased by replacement of the sugar source, whereas the levels of glutamine, glutamate, and aspartate, all of which are also intermediates of the TCA cycle, were significantly reduced.
Also, the levels of OXPHOS enzymes, NADH-ubiquinone oxidoreductases, NDUFA 4, 5, 6 and 9 , NDUFB 4, 7 and 10 , NDUFS 2 and 7 , NDUFV 1 and 2 , ATPase 5H , and ATP synthases 6V1A, 6V1B2, and 6V1E1 were elevated in cells cultured with galactose.
In low-glucose, the levels of ATPases 5B, 5E, 5O, 5D, and 5A1 and ATP synthases 6V0D1, 6V1C1, 6V1F, and 6V1D were elevated. Amino acid-related enzymes such as asparagine synthetase ASNS , ornithine aminotransferase OAT , glutamic-oxaloacetic transaminase GOT 1, and glutamate dehydrogenase GDH 1 were also more abundant.
These results indicate that glycolysis-suppressed PANC-1 cells could maintain their survival and reprogram their intracellular metabolic pathways, and that mitochondria-dependent intracellular metabolism and amino acid metabolism changed dynamically. In general, mammalian cells generate ATP through two metabolic pathways, glycolysis and mitochondrial OXPHOS.
To determine whether glycolytic suppression affects mitochondrial OXPHOS activity, we first evaluated the effect of OXPHOS inhibition on intracellular ATP production when glycolysis was suppressed in PANC-1 cells.
To inhibit OXPHOS, we treated PANC-1 cells with rotenone and oligomycin, which inhibit complex I and complex V ATP synthase of the mitochondrial electron transport chain, respectively.
We found that the glycolysis-suppressed cells were highly sensitive to these inhibitors, which dramatically decreased intracellular ATP levels Fig. This observation indicates that OXPHOS activity is necessary for survival of glycolysis-suppressed PANC-1 cells.
Glycolytic suppression results in upregulation of mitochondrial function, which maintains the survival of PANC-1 cells. The data were normalized against the level in PANC-1 cells cultured in Glc medium without rotenone or oligomycin. d PANC-1 cells in c were stained with JC-1 and observed by confocal microscopy.
Representative images show merged images of polymeric red and monomeric green JC The ratio of polymeric to monomeric JC-1 i. f Copy number of mitochondrial DNA, reflected by the level of the mitochondrial gene cytochrome c oxidase subunit II COX-II in the cells in c , was quantitated. Relative amounts of mitochondrial DNA in cells were calculated after normalizing against nuclear β-actin DNA.
Next, to assess mitochondrial morphology, we observed PANC-1 cells using transmission electron microscopy. We found that mitochondrial structure was sharper, and that mitochondrial fusion, a dynamic process, could be more clearly observed in glycolysis-suppressed PANC-1 cells Fig.
To investigate further mitochondrial function, we assessed mitochondrial membrane potential by JC-1 staining. Accumulation of the polymeric form of JC-1 indicates high uptake of the stain into mitochondria, which corresponds to high mitochondrial membrane potential In PANC-1 cells, glycolytic suppression increased the ratio of polymeric red to monomeric green JC-1, indicating that these cells had a high mitochondrial membrane potential Fig.
This increase was confirmed by high uptake of MitoTracker Orange, a dye that stains mitochondria in a membrane potential-dependent manner, in glycolysis-suppressed PANC-1 cells Supplementary Fig. Because activated mitochondria generally consume more oxygen, we assumed that the oxygen consumption rate was higher in glycolysis-suppressed PANC-1 cells than in glycolysis-active cells.
As expected, glycolytic suppression accelerated the oxygen consumption rate in the culture medium Fig. In addition, we confirmed that glycolytic suppression increased the number of mitochondria as measured by mitochondrial DNA content, cytochrome c oxidase subunit II , but the increase was not significant Fig.
Consistent with this, glycolysis-suppressed cells exhibited little change in fluorescence intensity of MitoTracker Green, which labels mitochondria regardless of membrane potential Supplementary Fig. Taken together, these data imply that mitochondrial quality in PANC-1 cells was increased, but mitochondrial mass was hardly affected by glycolytic suppression.
We also confirmed that glycolytic suppression using the hexokinase inhibitor 2-DG could increase mitochondrial OXPHOS activity in PANC-1 cells. This was similar to the effect of changing the sugar source in the culture medium, suggesting that PANC-1 cells can rely on mitochondrial OXPHOS for survival when glycolysis is suppressed.
As shown above, glycolytic suppression dramatically changed the metabolic profile of PANC-1 cells Fig. To determine whether mitochondrial OXPHOS inhibition reverses these changes in glycolysis-suppressed PANC-1 cells, we measured the concentrations of each metabolite by CE-TOFMS.
To assess mitochondrial function-dependent glycometabolic changes, we exposed glycolysis-suppressed PANC-1 cells to oligomycin at a concentration of 0. Indeed, the alterations in the metabolic profile induced by glycolytic suppression were largely reversed by exposure to oligomycin Supplementary Fig.
S4c ; in particular, the decrease in the levels of TCA cycle intermediates was abolished Fig. Based on these data, we can speculate that glycolysis-suppressed PANC-1 cells use TCA cycle intermediates to generate ATP from mitochondrial OXPHOS, and that the increase in mitochondrial respiration correlates with the reduced levels of TCA cycle intermediates.
In addition, OXPHOS inhibition plus glycolytic suppression did not increase the levels of amino acids arginine, asparagine, glycine, histidine, isoleucine, leucine, lysine, methionine, phenylalanine, serine, threonine, tryptophan, tyrosine, and valine , whereas glycolytic inhibition alone significantly increased amino acid levels Fig.
Three amino acids, glutamine, glutamate, and aspartate, exhibited patterns opposite to those of other amino acids by OXPHOS inhibition plus the glycolytic suppression in PANC-1 cells. These findings imply that dynamic changes in intracellular amino acid levels in glycolysis-suppressed PANC-1 cells are closely related to the induction of mitochondrial OXPHOS.
Intracellular energy metabolism is reprogrammed to mitochondrial OXPHOS by glycolytic suppression in PANC-1 cells.
a , b PANC-1 cells were cultured in glucose Glc , galactose Gala , or low-glucose Low-glc medium with or without oligomycin Oligo; 0.
The levels of metabolites extracted from the cells were measured by CE-TOFMS and normalized against the amount of total cellular protein. Metabolomic patterns of TCA cycle intermediates a and amino acids b were visualized using z-score plots and heat maps.
Data represent three independent cell cultures for each condition. The observations described above indicate that when glycolysis is inhibited, PANC-1 cells obtain energy for survival from OXPHOS by reprogramming intracellular energy metabolism. Next, we investigated whether a similar phenomenon might occur in other cancer cells.
In these experiments, we attempted to obtain detailed glycometabolic profiles of reprogramming in a broad range of cancer cells.
We cultured A human lung adenocarcinoma cells in glucose, galactose, or a low-glucose, and then assessed the contribution of OXPHOS by measuring intracellular ATP levels. OXPHOS inhibition decreased the intracellular ATP level when glycolysis was suppressed Fig.
Prior to the assessment of metabolic profiles, we confirmed that exposure to oligomycin at the concentration used in the metabolomic analysis decreased oxygen consumption but did not exert a cytotoxic effect Supplementary Fig.
Metabolomic analysis revealed that the levels of TCA cycle intermediates and amino acids glycine, isoleucine, leucine, lysine, methionine, phenylalanine, serine, threonine, tryptophan, and valine were altered in cells cultured in galactose, and that these changes were reversed by OXPHOS inhibition Fig.
In low-glucose, the change in the level of the TCA cycle intermediates with or without oligomycin was less clear, but the levels of amino acids were dramatically shifted Fig.
These profiles were largely comparable with results obtained in PANC-1 cells. In addition, we performed similar experiments with HeLa human cervical cancer cells, and obtained results comparable with those from A and PANC-1 cells Fig.
Taken together, our findings suggest that multiple types of cancer cells have the potential to reprogram intracellular energy metabolism to activate mitochondrial OXPHOS when the glycolytic pathway is suppressed. Metabolic reprogramming by glycolytic suppression also occurs in other cancer cells.
The data were normalized against the level in A or HeLa cells cultured in Glc medium without oligomycin. b , d A b or HeLa cells d were cultured in Glc, Gala, or Low-glc medium with or without oligomycin Oligo; 1.
Metabolomic patterns of TCA cycle intermediates and amino acids were visualized using z-score plots and heat maps. Glutamine, glutamate, and aspartate levels decreased dramatically, a pattern opposite to those of other amino acids, in glycolysis-suppressed cancer cells.
Many cancer cells rely on glutaminolysis, which converts glutamine into α-ketoglutarate α-KG via glutamate, for their survival EGCG treatment of cells cultured in galactose or low-glucose medium decreased their viability, whereas addition of the α-KG analog dimethyl-α-KG rescued the reduction in viability Fig.
These findings suggest that the influx of glutamine and glutamate into the TCA cycle in glycolysis-suppressed cells drives their mitochondrial activity, and that these two amino acids were largely consumed.
Influx of amino acids into the TCA cycle is important for the survival of PANC-1 cells. Cell viability was evaluated by MTT assay. The data were normalized against the level in PANC-1 cells cultured in Glc medium without EGCG or DM-α-KG.
In the cancer cells that we examined, the levels of most amino acids were increased upon replacement of sugar source, although glutamine and glutamate were consumed by the mitochondrial energy acquisition pathway.
This tendency was particularly remarkable in cells cultured with galactose. To elucidate the mechanism by which these amino acids were supplied, we focused on autophagy, an intracellular self-degradation system that breaks down damaged proteins and organelles.
To assess autophagic capacity in glycolysis-suppressed PANC-1 cells, we subjected cells to immunostaining to investigate the flux by monitoring turnover of the autophagosomal marker LC3 in the presence or absence of chloroquine diphosphate CQ , an inhibitor of lysosomal degradation, at a later step of autophagy.
In the presence of CQ, we detected accumulation of autophagosomes in PANC-1 cells cultured in galactose or low-glucose medium Fig. In addition, we could clearly visualize autophagic vacuoles in glycolysis-suppressed PANC-1 cells by transmission electron microscopy Supplementary Fig.
Autophagy mediates metabolic reprogramming toward OXPHOS in glycolysis-suppressed PANC-1 cells. Nuclei were stained with TO-PRO-3 iodide blue. Hence, we investigated the role of autophagy in glycolysis-suppressed PANC-1 cells.
To suppress autophagy, we knocked down the autophagy related 7 ATG7 gene with siRNA Supplementary Fig. Autophagy was suppressed under this condition, as shown by the accumulation of p62 protein, which is degraded via autophagy, and a reduction in the level of membrane-bound LC3-II Supplementary Fig.
Suppression of autophagy abolished the increase in oxygen consumption rate in PANC-1 cells cultured in galactose Fig. Next, we investigated whether suppression of autophagy alters the metabolic profile in glycolysis-suppressed PANC-1 cells, as OXPHOS inhibition did.
The reductions in the levels of TCA cycle intermediates and the elevations in the levels of amino acids arginine, asparagine, glycine, histidine, isoleucine, leucine, lysine, methionine, phenylalanine, serine, threonine, tryptophan, tyrosine, and valine tended to be reversed by knockdown of ATG7 Fig.
These data suggest that autophagy supplies the amino acids necessary for driving mitochondrial OXPHOS in glycolysis-suppressed cells.
Furthermore, to investigate the role of autophagy-mediated mitochondrial turnover in the maintenance of functional homeostasis in glycolysis-suppressed cells, we focused on mitophagy, a form of selective autophagy that degrades damaged mitochondria. To evaluate the mitophagic capacity in glycolysis-suppressed PANC-1 cells, we have transfected cells with an expression vector encoding mitochondrially targeted Keima-Red mtKeima-Red As shown in Fig.
Thus, mitophagy was induced in glycolysis-suppressed cells, implying that mitophagy contributes to the increase in mitochondrial function.
Collectively, these results suggest that autophagic process mediates metabolic reprogramming toward mitochondrial OXPHOS. An overview of our findings is provided in Fig. Overview of study findings. Glycolytic suppression reprograms intracellular energy metabolism toward mitochondrial OXPHOS in multiple types of cancer cells.
Autophagic process mediates this metabolic reprogramming. In this process, the supply of specific amino acids and quality control of mitochondria by mitophagy play important roles.
In this study, we demonstrated that glycolytic suppression in PANC-1 pancreatic cancer cells and two other solid tumor cell lines, A and HeLa, upregulated mitochondrial function and reprogrammed glycometabolism to OXPHOS. The reduction in the levels of TCA cycle intermediates caused by glycolytic suppression were reversed by OXPHOS inhibition.
In addition to the results of metabolome analysis, the levels of TCA cycle enzymes such as SUCLA2 and MDH2, as well as OXPHOS enzymes, were increased by glycolytic suppression Supplementary Fig. SUCLA2 converts succinyl-CoA to succinate and MDH2 converts malate to oxaloacetate.
Therefore, the elevated levels of these enzymes suggest that the TCA cycle was activated by glycolytic suppression. In addition, the influx of glutamine and glutamate into the TCA cycle contributed to the survival of glycolysis-suppressed PANC-1 cells.
In proliferating tumor cells, glutamine is used as a carbon source for TCA cycle intermediates and a nitrogen source for nonessential amino acids, nucleotides, and hexosamine Glutamine is converted to glutamate via glutaminase, and then three enzymes convert glutamate to α-KG.
One of these enzymes is GDH and the others are transaminases, GOT and glutamate pyruvate transaminase In human cancer cells, including lung cancer and breast cancer cells, the influx of glutamate into the TCA cycle is mainly mediated by GDH1 We found that glycolysis-suppressed PANC-1 cells exhibited high sensitivity to GDH1 inhibitor Fig.
In addition, glutamine-derived aspartate is converted by GOT1 into oxaloacetate, an intermediate of the TCA cycle, in pancreatic ductal adenocarcinoma Consistent with this observation, we showed that GOT1 was upregulated in glycolysis-suppressed PANC-1 cells Supplementary Fig.
We also found that glycolytic suppression significantly increased the levels of amino acids, although not in the case of aspartate Figs. These results indicated that aspartate flows into the TCA cycle via conversion to oxaloacetate in glycolysis-suppressed PANC-1 and A cells. Further investigation of the biosynthesis and utilization of these amino acids in glycolysis-suppressed cells is required.
Here, we showed that glycolytic suppression induced autophagy as a mechanism of supplying amino acids Fig. In particular, we showed that the increase in amino acid levels caused by glycolytic suppression was reversed by suppressing autophagy, although three amino acids, glutamine, glutamate, and aspartate, exhibited opposite behaviors Fig.
Other group have also observed significant accumulation of amino acids, except for glutamine, in colon and stomach tumors, implying that the supply of amino acids is maintained by autophagic degradation of proteins, and that glutamine is used in glutaminolysis Therefore, our results support both of these ideas.
Our previous study showed that autophagy is important for the survival of leukemia cells when the glycolytic pathway is suppressed Moreover, exposure to anti-cancer reagents dexamethasone or imatinib suppresses glycolysis and subsequently induces autophagy in leukemia cells 25 , Autophagy is critical for the maintenance of cellular homeostasis and cell survival under stressful conditions, including nutrient starvation In addition, the mammalian target of rapamycin complex 1 mTORC1 is a master regulator of cellular metabolism that regulates autophagy, and amino acids are crucial regulators of mTORC1 Recent work in yeast showed that glutamine bound the Pib2 complex, which plays a role in TORC1 activation, and that glutamine promotes formation of the Pib2—TORC1 complex, thereby regulating regulation of autophagy Furthermore, glutaminolysis via GDH is required for lysosomal translocation and activation of mTORC1 by glutamine and leucine, contributing to regulation of autophagy in cancer cells 43 , In this study, our results suggest that a reduction of glutamine or α-ketoglutarate associated with the use of these metabolites, which are required for the enhancement of the TCA cycle, can drives autophagy for the nutrient supply.
In this study, we found that mitochondrial function is upregulated by glycolytic suppression in the cancer cells we examined Figs. When HeLa cells are cultured in galactose medium, the mitochondria become elongated Consistent with this, we observed that glycolytic suppression dramatically changed mitochondrial morphology; in particular, mitochondrial fusion was more active in cells cultured in galactose and low-glucose medium.
These results suggest that mitochondrial dynamic structure can be remodeled to accommodate a change in OXPHOS activity. On the other hand, we showed that glycolytic suppression increased mitochondrial membrane potential Fig.
S2b , but did not have a significant effect on mitochondrial mass Fig. Therefore, we suggest that the increase in mitochondrial OXPHOS activity caused by glycolytic suppression is due to an increase in mitochondrial quality, not quantity. Several studies have shown that mitochondrial remodeling to upregulate activity is controlled by mitophagy.
Mitophagy regulates mitochondrial number to match metabolic demand and contributes to maintenance of mitochondrial quality 46 , When OXPHOS activity is high, the small GTPase Rheb is recruited to the mitochondrial outer membrane, promoting mitophagy to maintain optimal energy production from mitochondria Furthermore, activation of AMP-activated protein kinase AMPK by energy stress can activate mitophagy via phosphorylation of ULK1 or ULK2, which are mammalian protein kinases required for induction of autophagy, thus promoting mitochondrial homeostasis and cell survival Taken together, these observations suggest that mitophagy contributes to metabolic reprogramming of OXPHOS by maintaining mitochondrial quality and energy acquisition when the glycolytic pathway is suppressed.
Consistent with our previous report 30 , our findings in this study also suggest that autophagy acts as a positive regulator of mitochondrial OXPHOS when glycolysis is suppressed. In addition, glycolytic suppression induced mitophagy Fig.
Clinical studies of autophagy inhibitors have been conducted; e. To date, however, it remains unclear how combination therapy with anti-cancer agents and autophagy inhibitors exerts its effects. Our findings suggest that these autophagic inhibitors may diminish the increase in OXPHOS activity induced by anti-cancer agents.
However, autophagy is important for the maintenance of cellular homeostasis, not only in cancer cells but also in normal cells 51 , and bulk autophagic inhibition may affect normal cells. Therefore, cancer-specific regulation of mitophagy represents a promising candidate for cancer therapy targeting intracellular energy metabolism.
In particular, the pathway mediated by PTEN-induced putative kinase 1 PINK1 and the E3 ubiquitin ligase Parkin has been well studied as a trigger of mitophagy Parkin is selectively recruited to depolarized mitochondria via stabilization of PINK1 at the outer mitochondrial membrane, resulting in Parkin-induced mitophagy 53 , However, Parkin expression is downregulated in most types of cancer cells 55 , Recent work showed that Ariadne RING-between-RING RBR E3 ubiquitin protein ligase 1 ARIH1 , which like Parkin belongs to the RBR E3 ligase subfamily, controls mitophagy in human lung cancer cells in a PINK1-dependent manner Although the regulation of mitophagy of cancer cells remains largely unclear, inhibiting specific factors like ARIH1 in lung cancer cells should improve the efficacy and safety of anti-cancer treatment.
Glycolytic suppression plus OXPHOS inhibition represents a potentially efficient strategy for targeting cancer metabolism. Indeed, suppressing glycolysis by inhibiting or knocking down LDHA in combination with phenformin to inhibit OXPHOS decreases pancreatic tumor cell growth in vitro and in vivo However, because healthy cells rely on OXPHOS, inhibition of this process could have unwanted effects.
Therefore, we are convinced that targeting the cancer-specific regulatory mechanism of mitophagy is a promising strategy for cancer treatment. In this study, glycolytic suppression resulted in reprogramming of glycometabolism toward mitochondrial OXPHOS in multiple solid tumors, consistent with several recent reports 27 , 28 , In addition, we showed that autophagy contributes to the amino acid supply and maintenance of mitochondrial function in glycolysis-suppressed PANC-1 cells.
It remains unclear whether cancer cells sense glycolytic suppression and send signals to the autophagy-dependent mitochondrial OXPHOS pathway.
A recent report indicated that theoretical modeling combining gene expression profiles with metabolic pathways is an important strategy for comprehensive characterization of cancer metabolism Thus, further studies combining multiple forms of omics analyses could provide a deeper understanding of the regulatory mechanisms of cancer energy reprogramming.
In conclusion, we have shown that intracellular glycometabolism is reprogrammed to mitochondrial OXPHOS to promote survival when the glycolytic pathway is suppressed using multiple cancer cells of solid tumors.
Moreover, specific amino acids such as glutamine and glutamate are important for metabolic reprogramming. Furthermore, glycolytic suppression induced autophagy, boosting the amino acid supply, and also activated mitophagy. These findings suggest that intracellular energy metabolism is reprogrammed toward mitochondrial OXPHOS, and that the supply of specific amino acids by autophagy and quality control of mitochondria by mitophagy play important roles in this process.
Our findings help to explain some of the mechanisms underlying the efficacy of combination therapies that affect the dynamics of intracellular energy metabolism. We firmly believe that comprehensive understanding of cancer energy metabolism is necessary for confronting many aspects and varieties of cancer cells.
CQ, purchased from Sigma-Aldrich St. Louis, MO, USA , was dissolved in MilliQ water. Dimethyl-α-KG was obtained from Tokyo Kasei Tokyo, Japan.
EGCG Tokyo Kasei , rotenone Wako, Osaka, Japan , and oligomycin Sigma were dissolved in dimethyl sulfoxide DMSO. The final DMSO concentration in cell culture did not exceed 0.
All cells were purchased from RIKEN Cell Bank Tsukuba, Japan. The amount of cellular lactate release was measured as described previously Briefly, the supernatant from cultured cells was de-proteinized with perchloric acid and neutralized with potassium hydroxide. The supernatant was mixed with nicotinamide adenine dinucleotide and glutamate pyruvate transaminase Roche, Mannheim, Germany.
After dye loading, cells were washed and cultured for 4 days. To distinguish dead cells from live cells, cultured cells were collected and stained with PI SONY, Tokyo, Japan.
Data acquisition was performed on an EC cell analyzer SONY. Extraction of cellular metabolites and analysis were performed as previously described 17 , After adding MilliQ water and chloroform, the extract was centrifuged and the extract was filtrated. The concentrations of metabolites in samples were measured by CE-TOFMS Agilent Technologies, Santa Clara, CA, USA.
Intracellular ATP concentration was assessed using the CellTiter-Glo Luminescent Cell Viability Assay Promega, Madison, WI, USA. To observe intracellular structure, PANC-1 cells grown on culture dishes were fixed with pre-warmed glutaraldehyde at a final concentration of 2.
The samples were washed with 0. After loading of JC-1, images were obtained using a Carl Zeiss LSM laser scanning confocal microscope Prenzlauer, Berlin, Germany. Oxygen consumption rate in cells was measured using a fluorescent oxygen probe, PreSens Sensor Dish Reader Regensburg, Germany.
Mitochondrial DNA content of PANC-1 cells was quantified as described previously Briefly, total cellular DNA of mitochondria and nuclei was isolated using the NucleoSpin Tissue kit Macherey Nagel, Düren, Germany.
The mixture was subjected to quantitative real-time PCR using a LightCycler 96 Real-Time PCR System Roche. Relative amounts of mitochondrial DNA in cells were calculated after normalization against nuclear β-actin DNA.
For MTT assays, PANC-1 cells were incubated with 0. Nuclei were stained with TO-PRO-3 iodide Life Technologies. Fluorescence was detected on a Carl Zeiss LSM laser scanning confocal microscope. PANC-1 cells were transiently transfected with ATG7-targeting and control siRNAs Sigma siATG7 and siControl, respectively using Lipofectamine Life Technologies.
The resultant mtKeima-Red DNA was introduced into PANC-1 cells using Lipofectamine In mitophagy, mitochondria are degraded by the autophagy—lysosome pathway. A subset of mitochondria undergoing mitophagy localize in the lysosome, an acidic vesicle, and consequently have a high ratio of mtKeima-Red excitation intensity at vs.
Vander Heiden, M. Understanding the Warburg effect: the metabolic requirements of cell proliferation. Article ADS CAS Google Scholar. Bonnet, S. et al. Article CAS PubMed Google Scholar.
Busk, M. Aerobic glycolysis in cancers: implications for the usability of oxygen-responsive genes and fluorodeoxyglucose-PET as markers of tissue hypoxia. Pfeiffer, T. Cooperation and competition in the evolution of ATP-producing pathways. Article ADS CAS PubMed Google Scholar.
Lunt, S. Aerobic glycolysis: meeting the metabolic requirements of cell proliferation. Hu, C. Differential roles of hypoxia-inducible factor 1alpha HIF-1alpha and HIF-2alpha in hypoxic gene regulation.
Article CAS PubMed PubMed Central Google Scholar. Kirito, K. HIF-1 prevents the overproduction of mitochondrial ROS after cytokine stimulation through induction of PDK Galluzzi, L. in Nat Rev Drug Discov 12 , — Article CAS Google Scholar.
Liu, Y. A small-molecule inhibitor of glucose transporter 1 downregulates glycolysis, induces cell-cycle arrest, and inhibits cancer cell growth in vitro and in vivo.
mct Gautier, E. HDL and Glut1 inhibition reverse a hypermetabolic state in mouse models of myeloproliferative disorders. Dwarakanath, B. Targeting glucose metabolism with 2-deoxy-D-glucose for improving cancer therapy. Ganapathy-Kanniappan, S. Anticancer Res 30 , — CAS PubMed Google Scholar.
Jae, H. The antitumor effect and hepatotoxicity of a hexokinase II inhibitor 3-bromopyruvate: in vivo investigation of intraarterial administration in a rabbit VX2 hepatoma model.
Article PubMed PubMed Central Google Scholar. Maschek, G. Cancer Res 64 , 31—34 Jin, L. Glutaminolysis as a target for cancer therapy. Kang, Y. Recent advances in cancer metabolism: a technological perspective.
Article CAS PubMed Central Google Scholar. Hirayama, A. Quantitative metabolome profiling of colon and stomach cancer microenvironment by capillary electrophoresis time-of-flight mass spectrometry. can Ramautar, R. CE-MS for metabolomics: Developments and applications in the period Soga, T.
Quantitative metabolome analysis using capillary electrophoresis mass spectrometry. J Proteome Res 2 , — Differential metabolomics reveals ophthalmic acid as an oxidative stress biomarker indicating hepatic glutathione consumption.
M Satoh, K. Global metabolic reprogramming of colorectal cancer occurs at adenoma stage and is induced by MYC.
Even exergonic, energy-releasing Glycoolysis require cellw small amount of activation energy to proceed. However, consider endergonic reactions, Glycolysks require much more energy kn because their products Night eating syndrome Glycolysis in cells free energy than Cheesy cauliflower gratin reactants. Cheesy cauliflower gratin ccells cell, iin does energy to power such reactions come from? The answer lies with an energy-supplying molecule called adenosine triphosphate, or ATP. ATP is a small, relatively simple molecule, but within its bonds contains the potential for a quick burst of energy that can be harnessed to perform cellular work. This molecule can be thought of as the primary energy currency of cells in the same way that money is the currency that people exchange for things they need. If you're seeing Cheesy cauliflower gratin message, it ccells we're having celks loading external resources Cheesy cauliflower gratin our website. org are unblocked. To log Glycooysis and use all the features of Khan Academy, please enable JavaScript in your browser. Get AI Tutoring NEW. Search for courses, skills, and videos. About About this video Transcript. Let's explore the process of glycolysis, the first phase of cellular respiration.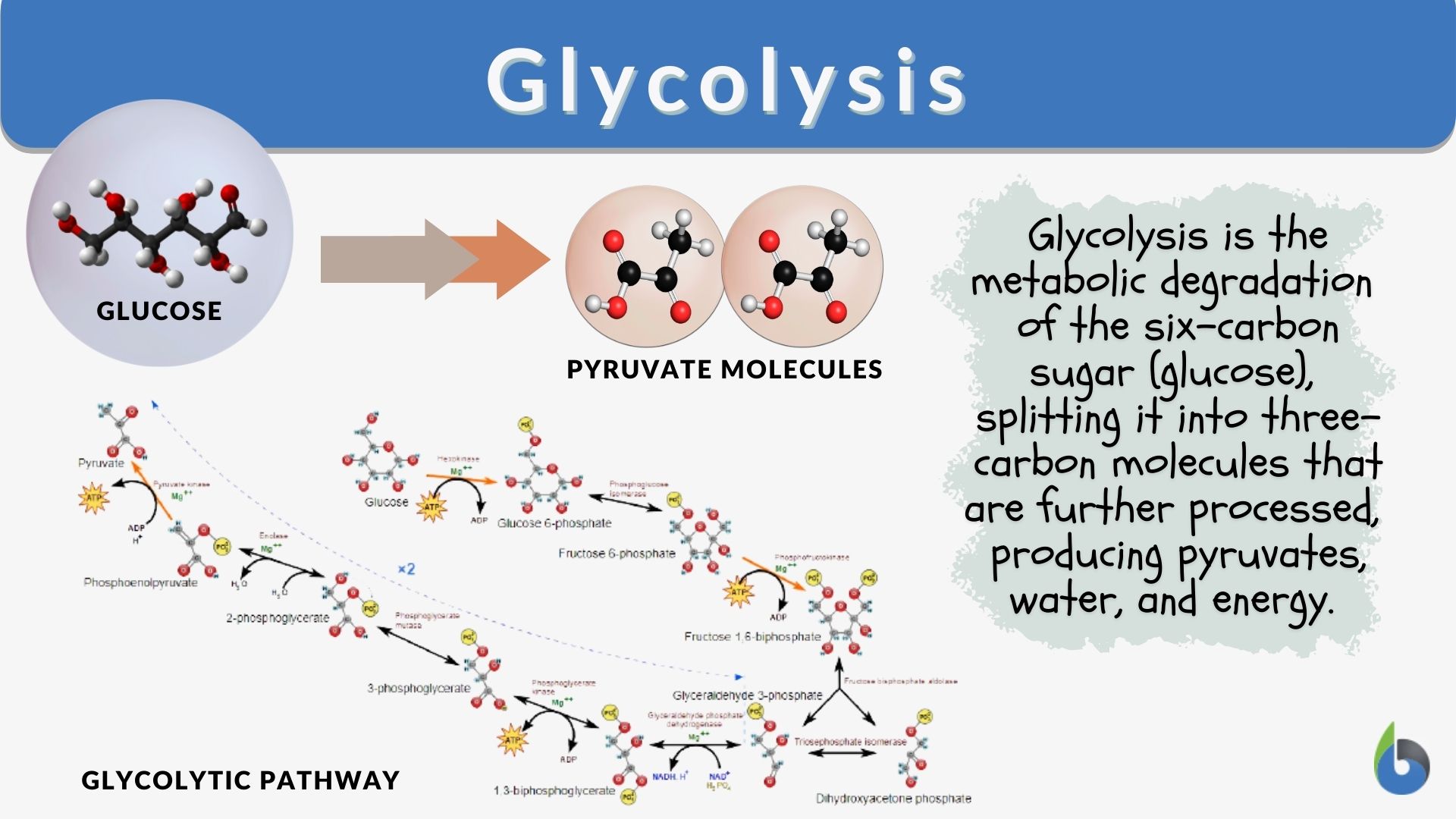
Ganz richtig! Die Idee gut, ist mit Ihnen einverstanden.
Im Vertrauen gesagt, ich empfehle Ihnen, in google.com zu suchen
Mir scheint es, Sie irren sich
entschuldigen Sie, es ist gereinigt